An overview of using Xenopus laevis as a model system.
Using tractable organisms (model systems) to answer fundamental questions in medicine and biology is a common practice since the ancient years. One of the model systems with important contributions is Xenopus laevis, the African clawed frog, a pseudotetraploid vertebrate which lives in fresh water. The reasons for its worldwide usage in research lie in the high degree of conservation of most essential cellular and molecular mechanisms; it is inexpensive, easily manipulated and large amounts of material can be readily obtained for a variety of experimental procedures. In this review, the material that is routinely obtained from Xenopus laevis, the most important experimental procedures, how the Xenopus system is used in research as well as the types of questions that can be answered only by using Xenopus are going to be discussed.
The breeding can be manipulated so that each frog can give eggs up to 3-4 times a year and the material used in research studies can vary from oocytes to cell-free extracts. The egg production is controlled by subcutaneous injections of 50-100 units of pregnant mare serum (PMS) in their dorsal lymph sacs several days before the second injection with 600-800 units of human chorionic gonadotropin (hCG) 12-16 hours before the desirable egg laying. Right after the second injection, they are placed in individual tanks and the next day the eggs are collected.
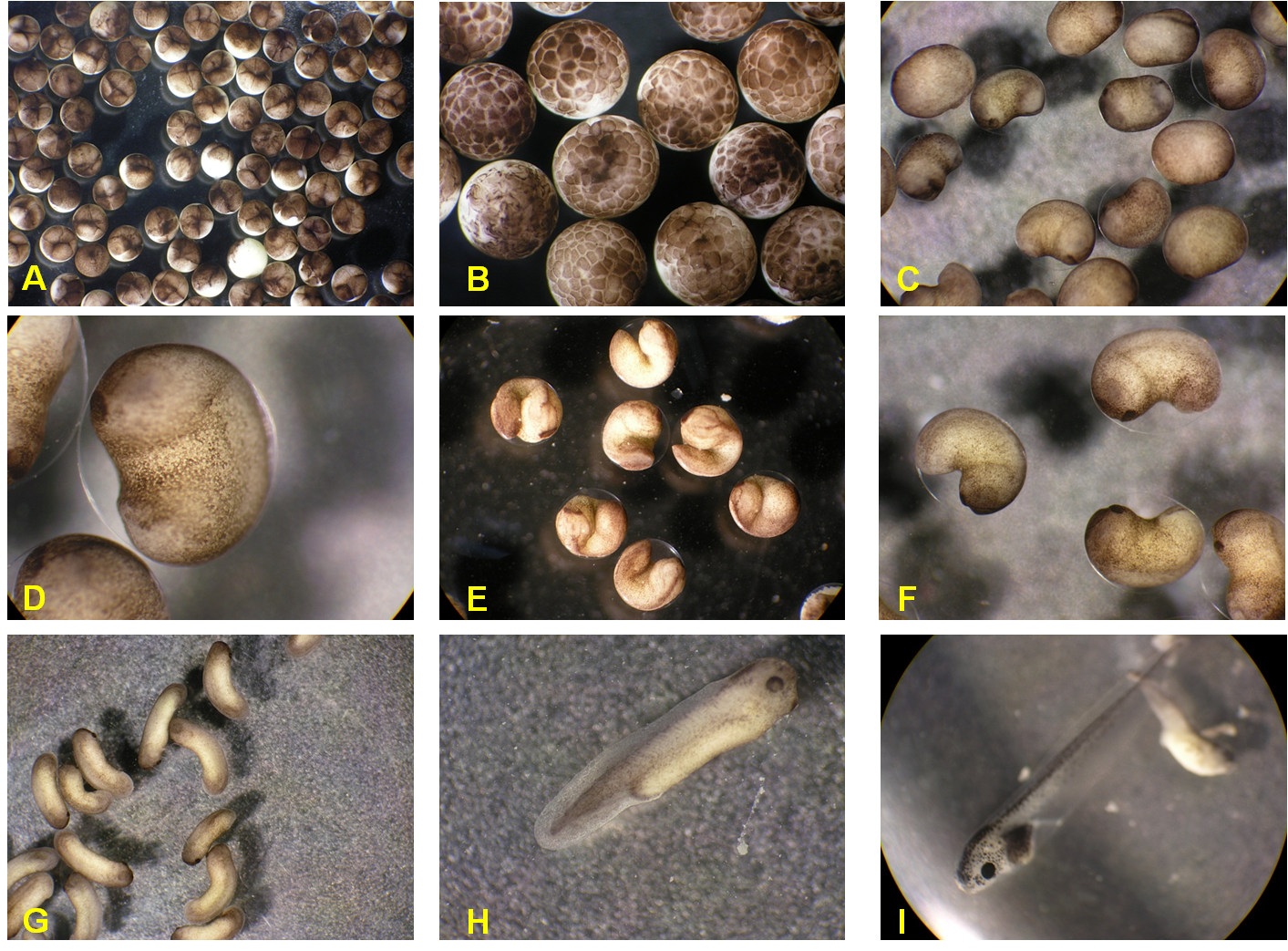
The oocytes are large cells of >1 mm in diameter. They contain a huge nucleus (or germinal vesicle), 100,000 times larger than a somatic cell nucleus, which occupies approximately the one-third of the oocyte’s volume and they develop rapidly. The vesicle is surrounded by a nuclear envelope with large pores that facilitate transportation from and to the cytoplasm. Admirably, the oocytes have synchronous cell cycle and they arrest at the first meiotic prophase until activation by progesterone. Progesterone activation results in meiotic maturation of the oocytes. The maturation stage is controlled by the activity of the maturation-promoting factor (MPF) [2], which is also known as p34cdc2 kinase and growth-associated histone H1 kinase. Upon progesterone activation and an increase of MPF activity, the nuclear structure collapses, the spindle is formed and the first meiotic division is completed. But the cycle is arrested again at the metaphase stage of the second meiotic cycle by the action of the cytostatic factor (CSF) and this signals the mature oocyte awaiting fertilization [3, 4]. After fertilization i.e., the merging of the sperm with the egg, the cytoplasmic pool of calcium is increased and this results in inactivation of both MPF and CSF, and release of the cell cycle (Figure 1) (see videos that show Xenopus development upon egg fertilization)
The material that Xenopus can offer in biology is great in terms of quality and quantity. For example, one frog ovary corresponds to 1000 mouse ovaries [5] and western blots can be performed with protein samples from just one oocyte (or 1 uL of extract – see below). Oocytes are very rich in all the necessary RNA and proteins for the first stages of development until early embryogenesis, i.e., the tadpole stage. They can contain up to 4 ug of ribosomal RNA, therefore each oocyte is able of synthesizing up to 400 ng of proteins per day. Single cell experimentation in Xenopus laevis oocytes is a routine for experimental biologists. In addition, they lack transcriptional activity until the mid-late blastula stage. It is estimated that the protein content is about 500 ug in each oocyte and approximately 10-50 mg/mL in the extract.
Xenopus laevis ovaries are large and transparent and may contain several hundreds of, also large, oocytes which are easily obtainable. Despite the difference in size, the processes of oogenesis and maturation are conserved in mammals. Therefore, they are extensively used by researchers studying the cell cycle.
The oocytes can be manually dissected using forceps from the ovaries or they can be extracted by digestion with collagenase (enzymatic defolliculation) of the four extracellular connective tissue layers that surround them. The advantages and disadvantages of either method have been discussed in detail in [6]. Then, from the oocytes, the nucleus can be manually removed. A pair of forceps with sharp tips is used to pierce a small opening on the cell and by squeezing force applied by another pair of forceps with blunt tips the nucleus is extracted [6, 7]. This results in oocytes without any nucleus (enucleated oocyte) and isolated nuclei. The experimental protocol with which the enucleation takes place is selected according to the downstream application, which can be immunoblotting [8], injection of a somatic nuclei and RNA synthesis for analysis of transcription [9], exogenous protein expression by injection of complementary RNA (cRNA) in the cytoplasm or of complementary DNA (cDNA) in the nucleus [10], injection of peptides or recombinant proteins [11] and others. Fully functional nuclei can be isolated and be used to study all nuclear processes including gene expression, chromatin dynamics, nuclear import and export of fluorescently labeled proteins or function of the nuclear pore complexes [12, 13].
The volume of one Xenopus laevis oocyte is approximately 1 uL. They can tolerate an injection of up to 50 nL using automatic nanoinjectors, without any adverse effect in the function. Several oocytes are arrayed onto special slides and sequentially injected with a nano-injector, as shown in this video.
Injections can be used for either gain-of-function (GOF) or loss-of-function (LOF) experiments or experiments involving both. By injections which contain any kind of DNA, RNA, plasmid DNA [14], morpholino oligonucleotides [15] or protein molecules, gene function can be easily manipulated. Several examples can be found in the literature; for example, the first experiments that led to the cloning of interferon [16], or the development of morpholino technology [17] were performed in Xenopus oocytes. Morpholino has been noted to generate high false positives [18]. Generation of transgenic Xenopus laevis embryos have also been described and have been used to assess gene expression involved in pathological diseases [19, 20]. Chromatin assembly can be studied in vivo in oocytes after injection of DNA into the germinal vesicle. Complementary strand synthesis or replication can be monitored by co-injection of radiolabeled dNTPs while nucleosome assembly can be assessed with supercoiling assay and micrococcal nuclease (MNase) assay. Virus replication is also possible after microinjection of viral RNA, which produces infectious viruses [21]. Apoptotic trigger waves were also identified through injection [22]. Li L et al injected morpholino oligos, TALEN mRNA, and full-length mRNAs to study the function of Etv6 [23]. Clairfeuille T et al injected cRNA for human and Blattella germanica Nav (synthesized through an mMessage mMachine kit from Thermo Fisher) into Xenopus laevis oocytes, sourced from either Xenopus one or Ecocyte, to study the inactivation of voltage-gated sodium channels [24].
As in other model systems used in developmental biology, transplantations can be easily performed in Xenopus oocytes. As explained above, due to the large oocytes’ size, enucleation is a routine procedure with Xenopus. An oocyte can be injected with nucleotides, such as morpholino or siRNA, which results in the loss of function of a target of interest. Then, the nucleus from this mutant oocyte or tadpole can be transferred into a ‘wild-type’ oocyte, whose nucleus has been removed or destroyed, and the healthy nucleus can be transplanted into a mutant oocyte and compared (Figure 2). These experimental procedures could replace strategies, such as the generation of transgenic clones, which are not very feasible in frogs yet. For more information read below the Gurdon experiment.
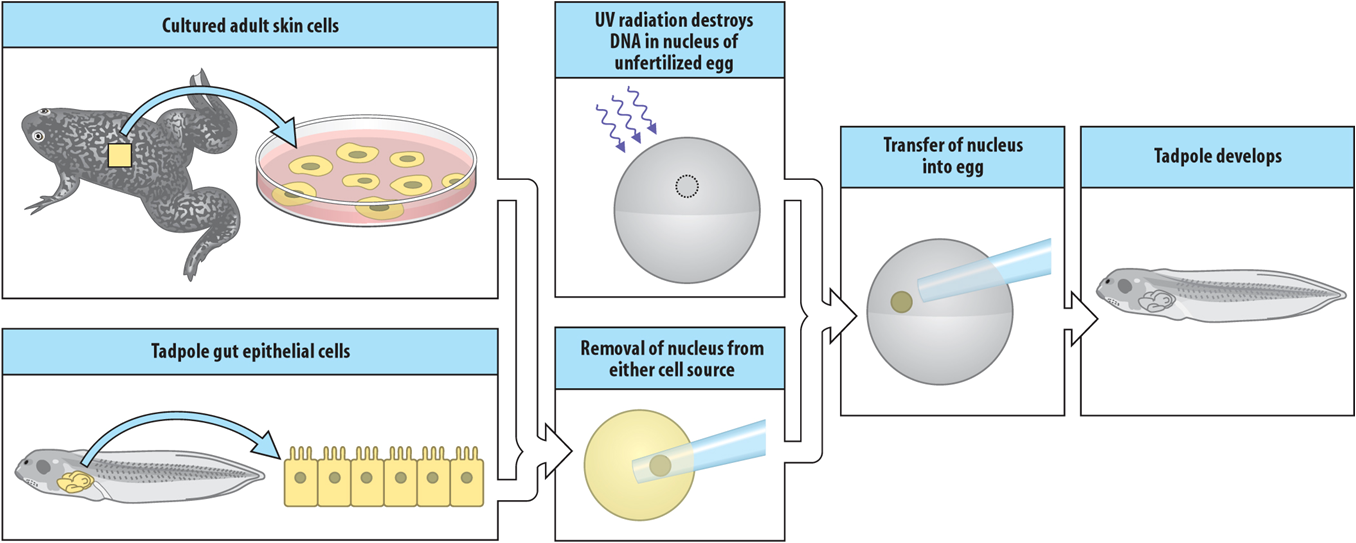
Furthermore, ion channels and membrane receptors of postmortem brains of humans with Alzheimer's disease were microtransplanted and were functional in Xenopus oocytes and have applications in studying channel activity related to human diseases [25-27]. Similarly, Xenopus oocytes are a great system for expression and study of neurotransmitters from human brain or cultured cells and its ligands (drugs, pesticides, etc) after injection of mRNA or cDNAs or membrane vesicles from native tissues. The currents generated in such experiments in oocytes are easily measured due to the large oocyte's size; hence the Xenopus system has significant contributions in the study of neurological disorders, such as epilepsy [28].
Chromatin assembly can be simply monitored after injection of exogenous proteins or after GOF or LOF experiments [29]. Similarly, proteins can be extracted for western blot analysis. Interestingly, chromatin immunoprecipitation (ChIP) is a technique used widely with cell lines to identify interplay between transcription factors or epigenetic markers with specific DNA elements, such as promoters. ChIPs can also be applied in Xenopus oocytes using the same principles but with much less starting material (10-15 oocytes) [30, 31]. There are cases where while Xenopus is not used, however, its chromatin components, such as histones, are examined [32].
Because of the large material that Xenopus oocytes and extracts offer, they have been used to make initial screens for small molecules that could be used in therapies of human diseases, such as cancer metastasis and generation of new blood vessels [33], protein degradation and DNA damage and repair [34, 35].
The large size of the oocyte opposes some problems in microscopy experiments (difficulty for antibodies to penetrate, background signal from the cortical pigment and the yolk platelets) rendering them less straightforward and requires optimization. Despite the difficulties, several protocols have been developed to study the localization and organization of the cytoskeleton and the cytoskeletal proteins (e.g., actin, tubulin, keratin) by immunofluorescence and confocal microscopy (for a full discussion see Becker and Gard, 2006 [36] ). Xenopus oocytes are also the preferred cells to study Ca2+ signaling.
The extract obtained from unfertilized Xenopus eggs is a cell-free system which supplements and sometimes overcomes the use of other model systems. It recapitulates all biological functions of the oocyte, and has been shown to self-organize into cell-like structures and undergo multiple rounds of division under the right conditions [37]. The extract is a widely used system to study the cell cycle in vitro, especially the processes of DNA replication and nucleus formation. These are very important processes for genome stability and any defect can contribute to numerous pathological conditions, such as cancer. Most, if not all, of the characteristics, are conserved from vertebrates to humans. Therefore, Xenopus egg extracts provide the simplicity to study vital cellular processes without the need to compensate for anything.
The first extracts were prepared by Lohka and Masui [38] and were widely used after Blow and Laskey and Hutchison et al in the mid-80s. These extracts could complete one cell cycle in vitro and could be stored frozen in liquid nitrogen and when fresh could exhibit several cell cycles [39, 40]. This cycle included the decondensation of the chromosomes, formation of the nuclear envelope, complete replication of DNA in a semi-conservative way as in humans, re-condensation of the chromosomes, break-down of the nuclear envelope, mitotic spindle formation and separation of the sister chromatids.
Since then, several variations of the original protocols for extract preparation exist from laboratory to laboratory according to available equipment and personal experience [1, 3, 41]. The differences are however small and all provide high-quality egg extracts that can be stored in liquid nitrogen for years.
Generally, the jelly coating of eggs is removed (dejellying) and the eggs are crushed by sequential centrifugations (Figure 3). When preferred, the unfertilized eggs are treated with calcium ionophore before crushing, which inactivates the cytostatic factor (CSF) and releases the cell cycle from the prophase into the next interphase in just a few minutes. The extracts obtained from these Ca2+-induced eggs are interphasic. Alternatively, the direct addition of calcium in the extract of uninduced eggs (CSF-arrested mitotic extract) has the same effect and results in the replication of sperm DNA. After the removal of debris, pigment granules, lipids and yolk proteins several types of extracts can be obtained depending on the scientific question to be studied (Figure 3).
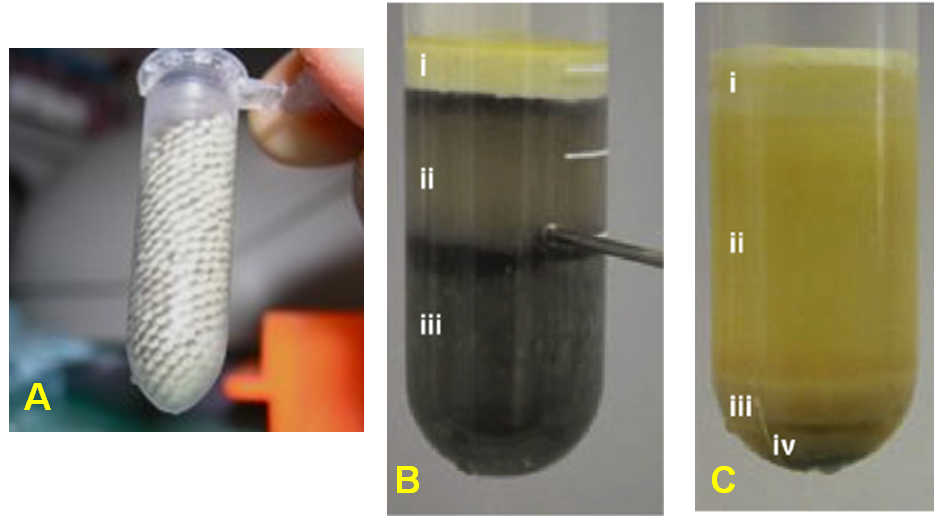
More detailed, low-speed extracts (LSE) contain the cytoplasm including light membranes, ribosomes and nuclear envelope and is prepared by medium-speed centrifugation (<60,000g) of CSF-arrested eggs. They can be either interphasic (from Ca2+-induced eggs as explained above) or mitotic (from non-induced eggs). LSE interphasic extracts demonstrate chromatin assembly, pre-replication complex (pre-RC) formation and loading on chromatin, origin firing and DNA replication, nuclear assembly while LSE mitotic extracts demonstrate chromosome condensation, mitotic spindle formation, and does not replicate DNA unless activated by Ca2+.
High-speed extracts (HSE) are prepared from LSE after additional high-speed centrifugation (100,000g) which separates the cytoplasm from membranes and ribosomes. Thus, HSE cannot form nuclei, DNA is assembled into chromatin and pre-RC is loaded, but double-stranded DNA cannot be replicated. However, it will synthesize the complementary strand when a single-stranded DNA molecule is added.
Transfection of DNA molecules in eukaryotic cells does not result in sufficient replication. On the other hand, Xenopus egg extracts are a powerful system, together with yeast, to study all stages of DNA replication, especially licensing of origins of replication and initiation, as they reproduce events in a semiconservative mechanism, like cells in vivo [42, 43]. In principle, an interphasic extract of good quality will start licensing the origins of DNA replication during the first minutes after the addition of an ATP regenerating mix and template DNA (demembranated sperm or plasmid DNA). DNA synthesis will start after 20-30 minutes and it will complete one round of DNA replication, it will fully decondense chromatin and will form fully functional nuclei in 60-80 minutes at 23oC (Figure 4C). Then, they enter mitosis, unless cycloheximide has been added to inhibit protein synthesis; thus, cyclin B is not synthesized to drive mitosis and the extract is arrested in a G2-like state.
Generally, progress through the cell cycle can be monitored by: immunofluorescence after the addition of biotin-labeled dUTPs (Figure 4E-F) or DNA replication assay after the addition of radiolabeled dNTPs and TCA precipitation (Figure 4D) [1].
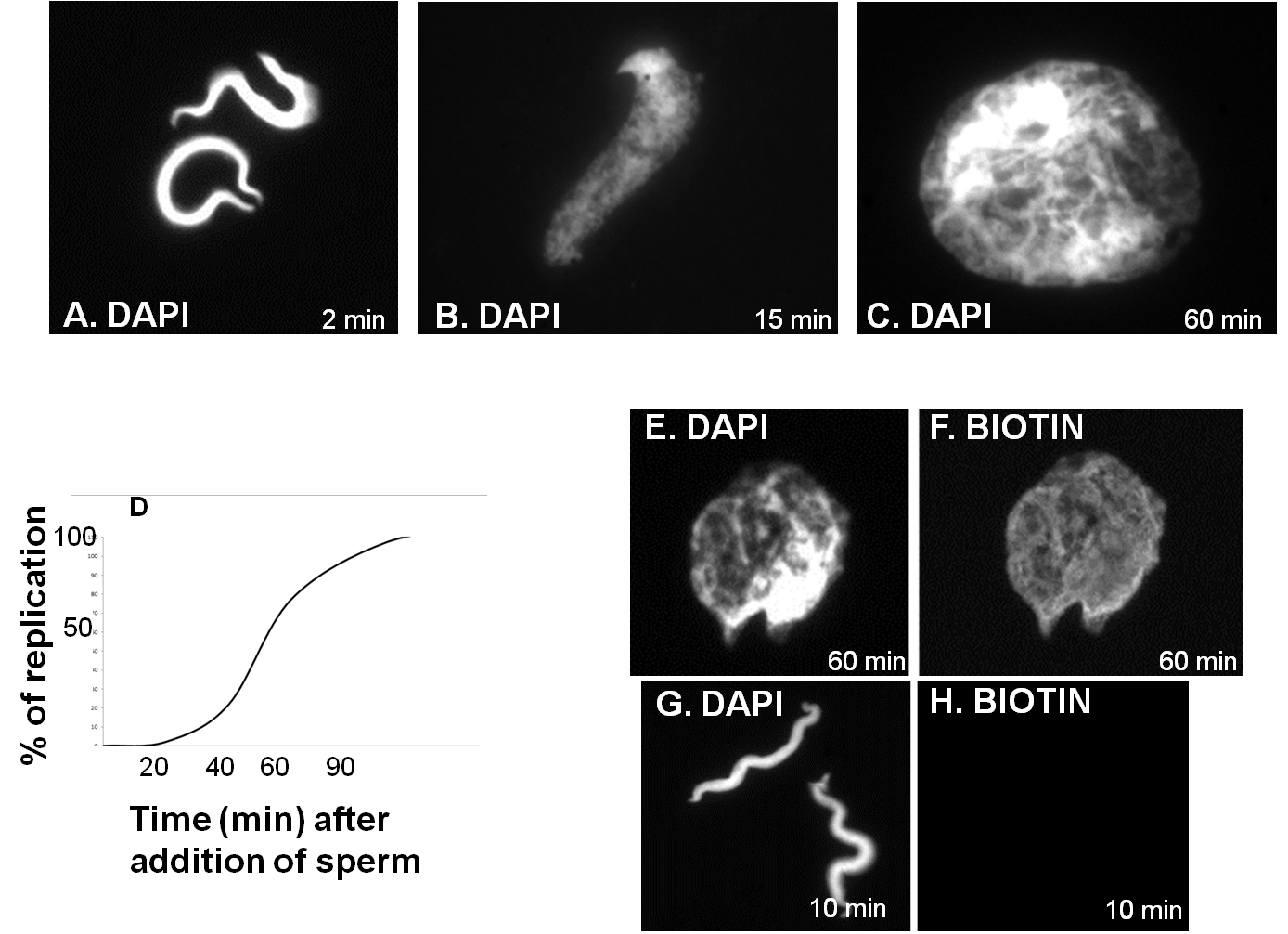
Since interphasic egg extracts do not have an assembled nucleus but contain all necessary components to construct one nucleus from scratch, they are an advantageous system to study nuclear assembly, nuclear pore and nuclear envelope formation. Using this to our advantage, adding a fluorescently labeled molecule in the extract, its transport from and to the nucleus can be easily studied. Likewise, the removal of a structural component of the nuclear envelope or nuclear pore could be used in such studies.
The great advantage of Xenopus extracts is the ease they provide in immunodepletion of particular components or addition of proteins, inhibitors or other molecules. These are standard procedures and there is no need for transfection or similar manipulations that are required, for example, with cultured cell lines. It is a simple addition in the tube. Besides, the cytosolic extract has no distinct cellular compartments formed and contains all proteins (e.g., cytosolic, nucleoplasmic, chromatin-bound) in one pool. Thus, the addition/depletion/inhibition of proteins is readily effective and the outcome of this manipulation easily measured [44]. For example, addition of high concentrations of okadaic acid, an inhibitor of protein phosphatases and especially protein phosphatase 2A (PP2A), directs the extract to bypass replication and to go directly to mitosis (Figure 5 the lower panel). Or immunodepletion of cyclin-dependent kinase 2, the major kinase in all stages of DNA replication, results in impaired replication as shown by a big reduction in the number of replication foci (Figure 5 the middle panel).
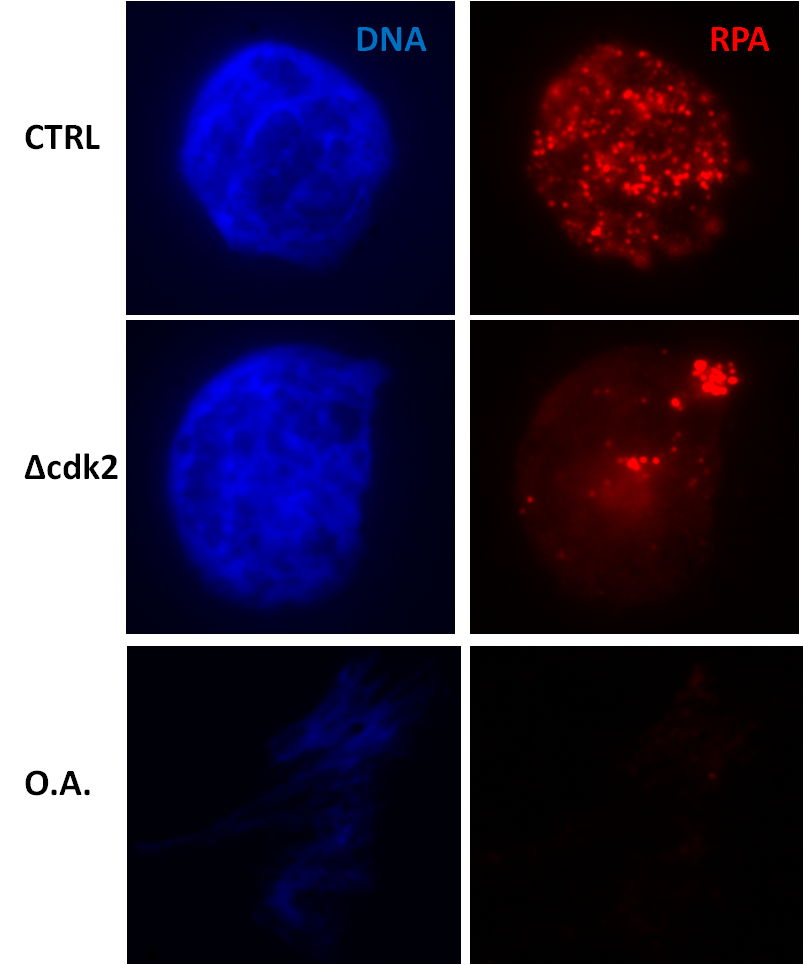
Xenopus egg extracts (as well as oocytes) provide an excellent environment to study posttranslational modifications (PTMs) of recombinant proteins. The proteins and signaling pathways in Xenopus show a high degree of conservation with humans [45]. Therefore, a recombinant protein can find its natural partners in an egg extract and thus retain full functionality. This is not always the case in bacteria or yeast, which do not have all the posttranslational modifications found in higher mammals. Egg extracts are highly concentrated compared to extracts from cultured cell lines (i.e. little material is required). The greatest advantage is that they offer a somewhat physiological context in either interphasic or mitotic egg extracts. Hence, when adding a binding partner as “bait” or a substrate of an enzyme in Xenopus extract, the probability to find the physiologically relevant interactors and upstream or downstream effectors at the appropriate stage of the cell cycle is very high.
Epigenetics and histone modifications are considered important for embryonic development and the route of several pathological conditions, including cancer. Chromatin of interphasic or mitotic Xenopus egg extracts can be readily purified at various stages (e.g., pre-licensing of replication origins, DNA replication firing, late S-phase, etc) through a sucrose gradient and compared. Purified chromatin can be assessed by immunoblotting, immunofluorescence, etc. Xenopus offers the ability to identify novel PTMs that are difficult to find in more complex samples, such as cell lines. Several other resources for genetic, genomic and proteomic studies can be found here [46].
Xenopus model system has numerous other applications, such as the understanding of regeneration [47, 48], microtubule nucleation [49], phase separation [50], brain development [15] and many others. Other areas of research in which Xenopus embryos have significant contribution are the eye and vision research, heart development, immunology. Based on embryological analysis of Xenopus eye after manipulation of gene expression specifically in the eye by microinjections of the blastomeres, several genes and signaling pathways have been uncovered crucial for eye development and retina fate (Xenopus White Paper 2009). In terms of gene expression, Mughal BB et al have identified reference genes such as clta.L, sub1.L etc. for quantitative real-time PCR studies in developing Xenopus laevis [51]. DeLay BD et al established the procedure for kidney-specific knockout of lhx1 gene with CRISPR/Cas9 in the allotetraploid frog [52].
In conclusion, the main advantages of the Xenopus system are the large oocytes and cell-free extracts, which offer plenty of material (protein, DNA, RNA, etc) for biochemical studies and easiness in microinjection, cell cycle synchrony, and conserved molecular mechanisms. Given that embryonic development shares many characteristics with human cancers, Xenopus laevis and X. tropicalis are great tools to not only study tumorigenesis but also to answer general fundamental questions in biology and medicine [45, 53].
Xenopus laevis has become one of the most promising models to study the mechanisms of regeneration. Human tissue does not have the regenerative capacity of the amphibian skin and develops fibrosis after dermal injury. Therefore, the analysis of Xenopus regenerative blastemal cells and comparison with myofibroblast-mediated scarring in humans would help to understand regenerative processes in humans. Aztekin C et al identified a regeneration-organizing cell in the Xenopus tail using single-cell RNA-seq [54]. For example, a recent study used Xenopus cells labeled with infrared laser‐evoked gene operator (IR-LEGO) in the study of skin regeneration [55, 56]. In addition, the genome sequencing in Xenopus laevis has already been finalized and can be applied to the gene expression profiling in the amphibian regenerative cells [57]. Furthermore, another recent publication presented several assays to study cellular and molecular wound-healing mechanisms in Xenopus embryos [58]. The described methods included generation of knockdown and overexpression constructs and incorporation of mRNAs encoding fluorescent markers.
Regeneration of central and peripheral structures of the nervous system is another area of research, where Xenopus laevis has been proven to be an effective study model. The use of transgenic Xenopus for studies of axon regeneration includes the introduction of one or two labeling molecules, such as dextran amines, to follow the regrowth of the experimentally damaged axons [59]. Another protocol, which uses Xenopus to investigate the regeneration of spinal cord, has recently been published [60]. The study is based on the ability of these amphibian species to regenerate the spinal cord at larval stage of development. In addition, a recent study has applied quantitative proteomics to analyze the variety of proteins expressed in Xenopus spinal cord in regenerative and nonregenerative stages [61]. With regard to the visual sensory system, Xenopus has been used to study the regeneration of eye structures, such as lens and retina [62, 63].
Xenbase, the Xenopus model organism database, is a vital resource for all researchers [64-66]. It contains genomic, mRNA, proteomic and functional annotations and is directly linked to several databases, such as NCBI or Uniprot. Xenopus laevis genome has been only recently sequenced fully. Initially, at the beginning of the 2000s, the tetraploid genome was thought to bring about problems that would not be observed if the diploid Xenopus tropicalis was chosen for sequencing. Indeed, the knowledge obtained from X. tropicalis genome as well as the recent advances in DNA sequencing helped to have Xenopus laevis genome fully assembled and sequenced. Therefore, proteomics studies can be routinely performed [67-71]. Genes can also be obtained from Source Biosciences [50].
Zoologist and environmental biologist Thomas H. Morgan ( Nobel Prize in Physiology or Medicine in 1933) was one of the first to use Xenopus, before he moved to study Drosophila. But it was endocrinologist Lancelot Hogben's work that established Xenopus as a model system. Until then, the breeding could not be manipulated and therefore experiments could be performed only in late winter to spring during which the natural breeding of frogs happens. His work led to the development of the most reliable and rapid pregnancy test until the 1960s, as he discovered that when urine from pregnant women was injected into female Xenopus laevis, the frog laid eggs indicating the presence of human chorionic gonadotropin (hCG) in the urine [5, 72] (see also http://www.columbia.edu/cu/biology/faculty/kelley/webessay/histories.html). Therefore, after a simple injection of hCG, Xenopus laevis lays a huge number of eggs every 3-4 months anytime in a year, which can be exploited by biochemists, molecular or developmental biologists. The eggs can be used directly as single cell oocytes or, after a simple experimental procedure, egg extracts can be prepared.
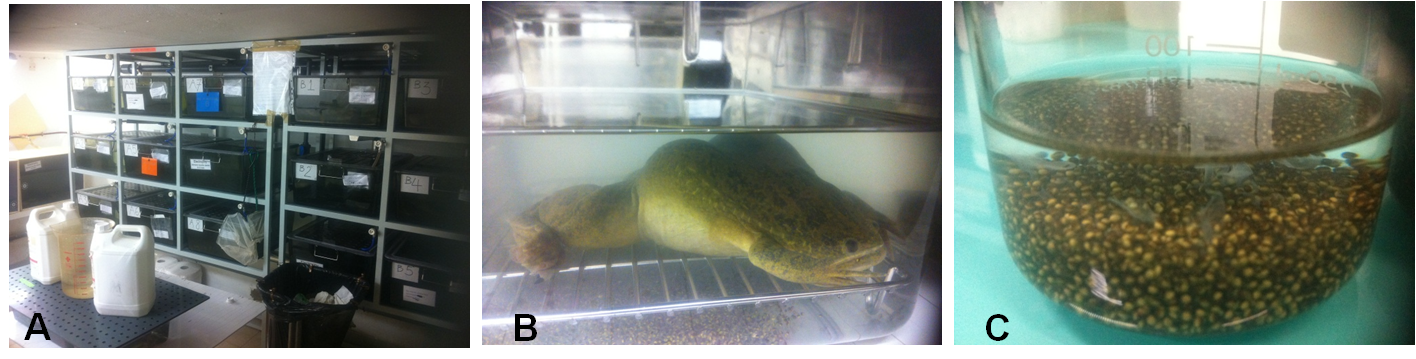
Later, Michaïl Fischberg and his colleagues found one frog in their colonies producing diploid embryos with one nucleolus (instead of two), and they generated the homozygous frog which laid the foundation for nuclear transplantation, as this O-nu mutation could be used as a genetic marker of nuclear transplantation [73]. A few years later, experimental biologist John Gurdon (M. Fischberg’s student) discovered that the O-nu mutation was the result of a complete deletion of several ribosomal genes [74]. For example, the concept that differentiated cells can be reprogrammed to become pluripotent was initially introduced in 1962 by Gurdon, who took the nucleus of a mature intestinal cell of a tadpole and placed it in an egg cell, whose nucleus had been removed. He then saw that this egg developed into a healthy tadpole [75]. For his groundbreaking work on cell reprogramming, Gurdon was awarded the Nobel Prize in Physiology or Medicine in 2012, jointly with S. Yamanaka.
Since then (the 1960s), even though Xenopus’ natural environment is restricted to South Africa, it has become a very popular model system for a variety of studies and is now a common inhabitant of research laboratories worldwide. Xenopus is a remarkable, widely used model system for biology and medicine. In the laboratory environment, the frogs are usually housed in plastic tanks with tap water in groups of 6-15 with a plastic pipe inside the tank which would provide a hiding place for the frogs. The temperature should be around 16-20°C and though wild frogs live in somewhat dark environments, a circle of 12-14 hours of light with 10-12 hours of dark is vital for good health (Figure 6).
- Wasserman W, Masui Y. A cytoplasmic factor promoting oocyte maturation: its extraction and preliminary characterization. Science. 1976;191:1266-8 pubmed
- Murray A. Cell cycle extracts. Methods Cell Biol. 1991;36:581-605 pubmed
- Rasar M, Hammes S. The physiology of the Xenopus laevis ovary. Methods Mol Biol. 2006;322:17-30 pubmed
- Gurdon J. From nuclear transfer to nuclear reprogramming: the reversal of cell differentiation. Annu Rev Cell Dev Biol. 2006;22:1-22 pubmed
- Liu X, Liu X. Oocyte isolation and enucleation. Methods Mol Biol. 2006;322:31-41 pubmed
- Lehman C, Carroll D. Isolation of large quantities of functional, cytoplasm-free Xenopus laevis oocyte nuclei. Anal Biochem. 1993;211:311-9 pubmed
- Gall J, Murphy C, Callan H, Wu Z. Lampbrush chromosomes. Methods Cell Biol. 1991;36:149-66 pubmed
- Ford C, Gurdon J. A method for enucleating oocytes of Xenopus laevis. J Embryol Exp Morphol. 1977;37:203-9 pubmed
- Altafaj X, Joux N, Ronjat M, De Waard M. Oocyte expression with injection of purified T7 RNA polymerase. Methods Mol Biol. 2006;322:55-67 pubmed
- Zagotta W, Hoshi T, Aldrich R. Restoration of inactivation in mutants of Shaker potassium channels by a peptide derived from ShB. Science. 1990;250:568-71 pubmed
- Maco B, Fahrenkrog B, Huang N, Aebi U. Nuclear pore complex structure and plasticity revealed by electron and atomic force microscopy. Methods Mol Biol. 2006;322:273-88 pubmed
- Reynolds F, Premkumar E, Pitha P. Interferon activity produced by translation of human interferon messenger RNA in cell-free ribosomal systems and in Xenopus oöcytes. Proc Natl Acad Sci U S A. 1975;72:4881-5 pubmed
- Heasman J, Kofron M, Wylie C. Beta-catenin signaling activity dissected in the early Xenopus embryo: a novel antisense approach. Dev Biol. 2000;222:124-34 pubmed
- Ogino H, McConnell W, Grainger R. High-throughput transgenesis in Xenopus using I-SceI meganuclease. Nat Protoc. 2006;1:1703-10 pubmed
- Gamarnik A, Andino R. Replication of poliovirus in Xenopus oocytes requires two human factors. EMBO J. 1996;15:5988-98 pubmed
- Dascal N. The use of Xenopus oocytes for the study of ion channels. CRC Crit Rev Biochem. 1987;22:317-87 pubmed
- Miledi R, Dueñas Z, Martinez Torres A, Kawas C, Eusebi F. Microtransplantation of functional receptors and channels from the Alzheimer's brain to frog oocytes. Proc Natl Acad Sci U S A. 2004;101:1760-3 pubmed
- Buckingham S, Pym L, Sattelle D. Oocytes as an expression system for studying receptor/channel targets of drugs and pesticides. Methods Mol Biol. 2006;322:331-45 pubmed
- Roche D, Almouzni G, Quivy J. Chromatin assembly of DNA templates microinjected into Xenopus oocytes. Methods Mol Biol. 2006;322:139-47 pubmed
- Kuo M, Allis C. In vivo cross-linking and immunoprecipitation for studying dynamic Protein:DNA associations in a chromatin environment. Methods. 1999;19:425-33 pubmed
- Stewart D, Tomita A, Shi Y, Wong J. Chromatin immunoprecipitation for studying transcriptional regulation in Xenopus oocytes and tadpoles. Methods Mol Biol. 2006;322:165-81 pubmed
- Becker B, Gard D. Visualization of the cytoskeleton in Xenopus oocytes and eggs by confocal immunofluorescence microscopy. Methods Mol Biol. 2006;322:69-86 pubmed
- Lohka M, Masui Y. Formation in vitro of sperm pronuclei and mitotic chromosomes induced by amphibian ooplasmic components. Science. 1983;220:719-21 pubmed
- Blow J, Laskey R. Initiation of DNA replication in nuclei and purified DNA by a cell-free extract of Xenopus eggs. Cell. 1986;47:577-87 pubmed
- Hutchison C, Cox R, Drepaul R, Gomperts M, Ford C. Periodic DNA synthesis in cell-free extracts of Xenopus eggs. EMBO J. 1987;6:2003-10 pubmed
- Laskey R, Gurdon J. Induction of polyoma DNA synthesis by injection into frog-egg cytoplasm. Eur J Biochem. 1973;37:467-71 pubmed
- Klein S, Gerhard D, Wagner L, Richardson P, Schriml L, Sater A, et al. Resources for genetic and genomic studies of Xenopus. Methods Mol Biol. 2006;322:1-16 pubmed
- Bowes J, Snyder K, Segerdell E, Gibb R, Jarabek C, Noumen E, et al. Xenbase: a Xenopus biology and genomics resource. Nucleic Acids Res. 2008;36:D761-7 pubmed
- Gurdon J, Hopwood N. The introduction of Xenopus laevis into developmental biology: of empire, pregnancy testing and ribosomal genes. Int J Dev Biol. 2000;44:43-50 pubmed
- Gurdon J, ELSDALE T, Fischberg M. Sexually mature individuals of Xenopus laevis from the transplantation of single somatic nuclei. Nature. 1958;182:64-5 pubmed
- Brown D, Gurdon J. ABSENCE OF RIBOSOMAL RNA SYNTHESIS IN THE ANUCLEOLATE MUTANT OF XENOPUS LAEVIS. Proc Natl Acad Sci U S A. 1964;51:139-46 pubmed
- Gurdon J. The developmental capacity of nuclei taken from intestinal epithelium cells of feeding tadpoles. J Embryol Exp Morphol. 1962;10:622-40 pubmed
- Materials and Methods [ISSN : 2329-5139] is a unique online journal with regularly updated review articles on laboratory materials and methods. If you are interested in contributing a manuscript or suggesting a topic, please leave us feedback.
- gene