- 1Department of Applied Genetics and Cell Biology, University of Natural Resources and Life Sciences, Vienna, Austria
- 2Department of Chemistry, University of Natural Resources and Life Sciences, Vienna, Austria
N-glycosylation is an essential protein modification that plays roles in many diverse biological processes including protein folding, quality control and protein interactions. Despite recent advances in characterization of the N-glycosylation and N-glycan processing machinery our understanding of N-glycosylation related processes in plant development is limited. In Arabidopsis thaliana, failure of mannose trimming from oligomannosidic N-glycans in the endoplasmic reticulum (ER) and cis/medial-Golgi leads to a defect in root development in the mns123 triple mutant. Here, we show that the severe root phenotype of mns123 is restored in asparagine-linked glycosylation (ALG)-deficient plants with distinct defects in the biosynthesis of the lipid-linked oligosaccharide precursor. The root growth of these ALG-deficient plants is not affected by the α-mannosidase inhibitor kifunensine. Genetic evidence shows that the defect is uncoupled from the glycan-dependent ER-associated degradation (ERAD) pathway that removes misfolded glycoproteins with oligomannosidic N-glycans from the ER. Restoration of mannose trimming using a trans-Golgi targeted α-mannosidase suppresses the defect of mns123 roots. These data suggest that processing of terminal mannose residues from oligomannosidic N-glycans is important for an unknown late-Golgi or post-Golgi process that is implicated in proper root formation.
Introduction
N-glycosylation of proteins is an essential co- and posttranslational modification in eukaryotes. During N-glycosylation a preassembled lipid-linked oligosaccharide is transferred en bloc to an asparagine residue that is present in the consensus sequence motif Asn-X-Ser/Thr of a polypeptide (Aebi, 2013). Assembly of the lipid-linked oligosaccharide occurs in an ordered stepwise manner by ALG (asparagine linked glycosylation) enzymes. The first steps of the lipid-linked oligosaccharide biosynthesis take place on the cytosolic side of the endoplasmic reticulum (ER) membrane. The synthesized Man5GlcNAc2-dolichol pyrophosphate is transported across the ER membrane and used by a series of different ALGs as an acceptor substrate. In the ER lumen, the first mannose residue is transferred by the α1,3-mannosyltransferase ALG3. Three additional mannose residues are attached to the B- and C-branches of the lipid-linked precursor by the α1,6-mannosyltransferase ALG12 and the α1,2-mannosyltransferase ALG9 (Figures 1A,B). The biosynthesis of the dolichol-linked oligosaccharide is completed by the successive addition of three glucose residues catalyzed by the glucosyltransferases ALG6, ALG8, and ALG10. The oligosaccharyltransferase complex transfers the fully assembled oligosaccharide to asparagine residues of newly synthesized proteins (Strasser, 2016).
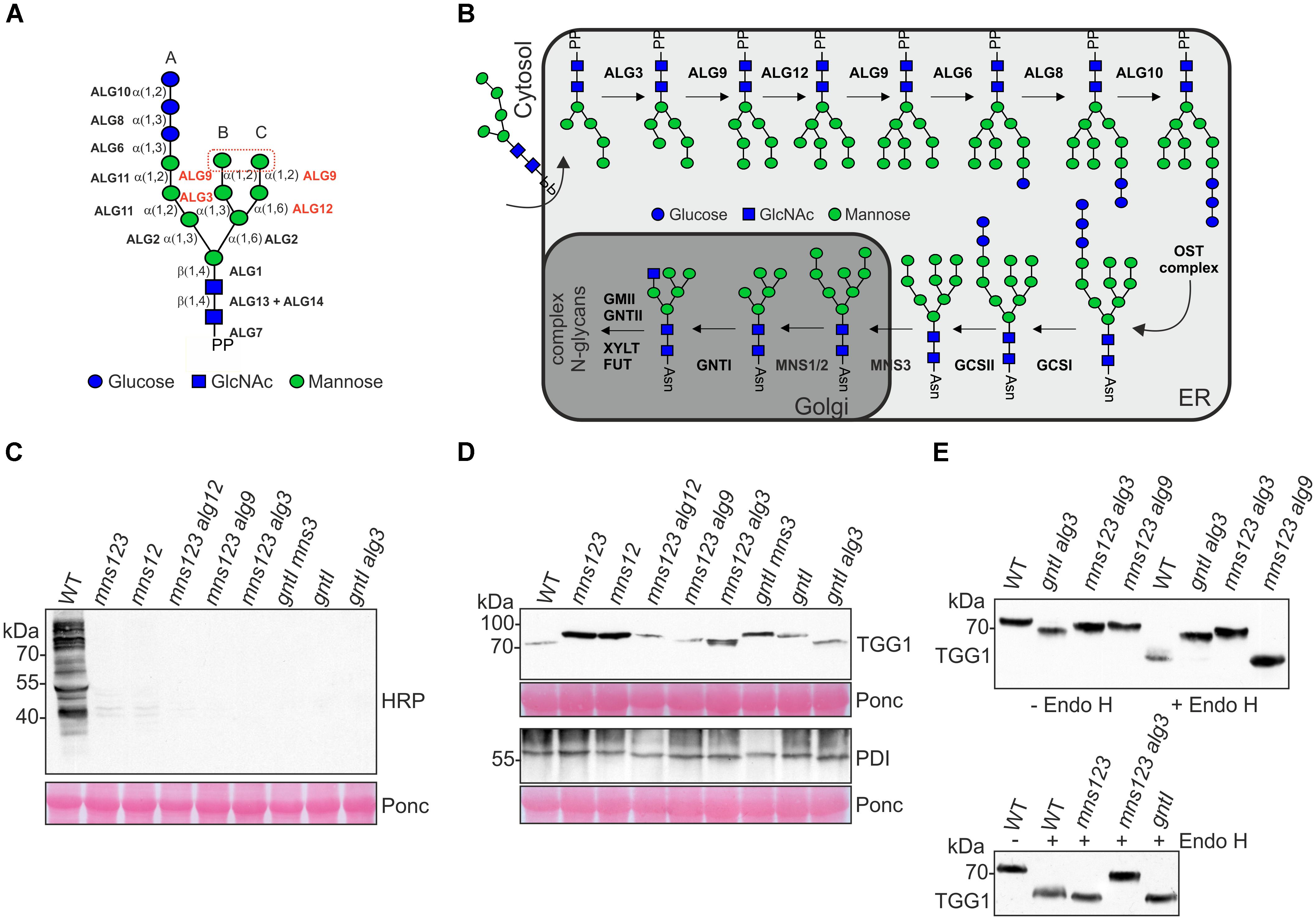
Figure 1. (A) Schematic illustration of the preassembled dolichol pyrophosphate-linked oligosaccharide precursor. The ALG glycosyltransferases required for the precursor biosynthesis are shown. The ALGs involved in the transfer of mannose residues in the lumen of the ER and the two terminal mannose residues on the B- and C-branches are highlighted in red. The symbol representation for glycans is drawn according to the guidelines from the Consortium for Functional Glycomics. (B) Illustration of N-glycan biosynthesis and processing pathways in wild-type. For the biosynthesis pathway only the steps in the lumen of the ER are shown. The following abbreviations are used for the enzymes: ALG3, α1,3-mannosyltransferase; ALG6, α1,3-glucosyltransferase; ALG8, α1,3-glucosyltransferase; ALG9, α1,2-mannosyltransferase; ALG10, α1,2-glucosyltransferase; ALG12, α1,6-mannosyltransferase; OST, oligosaccharyltransferase; GCSI, α-glucosidase I; GCSII, α-glucosidase II; MNS3, ER-α-mannosidase I; MNS1/MNS2, Golgi-α-mannosidase I; GNTI, N-acetylglucosaminyltransferase I; GMII, Golgi α-mannosidase II; GNTII, N-acetylglucosaminyltransferase II; XYLT, β1,2-xylosyltransferase; FUT, core α1,3-fucosyltransferase. Asn, asparagine of the N-glycosylation site (consensus: Asn-X-Ser/Thr). PP, dolichol pyrophosphate. (C) Immunoblot analysis with antibodies against complex N-glycans. A positive signal is only observed in wild-type Col-0 (WT) carrying processed complex N-glycans with β1,2-xylose and core α1,3-fucose residues. Ponceau S (Ponc) staining of the membrane is shown as a loading control. (D) Immunoblot analysis with antibodies directed against the glycoproteins TGG1 or PDI. (E) Endo H digestion of protein extracts and immunoblot analysis.
N-glycan processing in the ER starts by removal of terminal glucose residues from the transferred oligosaccharide. The oligomannosidic N-glycans are trimmed further by specific α-mannosidases (MNS1, MNS2, and MNS3). MNS3 displays ER-α-mannosidase I activity and cleaves a single mannose residue from the B-branch of the oligomannosidic N-glycan (Figure 1A). The functionally redundant Golgi-α-mannosidases MNS1 and MNS2 act downstream of MNS3 and remove three mannose residues from the A- and C-branches (Figure 1B) (Liebminger et al., 2009). The resulting N-glycan (Man5GlcNAc2) is used by N-acetylglucosaminyltransferase I (GNTI) for the initiation of complex N-glycan processing in the cis/medial-Golgi (von Schaewen et al., 1993; Strasser et al., 1999).
In Arabidopsis thaliana, it is well documented that mutants deficient in the transfer of the lipid-linked oligosaccharide precursor or the first N-glycan processing reactions in the ER are lethal (Boisson et al., 2001; Gillmor et al., 2002; Koiwa et al., 2003; Lerouxel et al., 2005; Soussillane et al., 2009; Farid et al., 2011; Jeong et al., 2018). Moreover, N-glycan processing reactions in the Golgi apparatus generate complex N-glycans that are crucial for salt stress tolerance in Arabidopsis (Kang et al., 2008) and severely affect plant growth and reproduction in Oryza sativa (Fanata et al., 2013; Harmoko et al., 2016) and Lotus japonicus (Pedersen et al., 2017). Disruption of MNS1 to MNS3 genes in the Arabidopsis mns123 triple mutant results in a severe vegetative growth defect with the formation of short and radially swollen roots (Liebminger et al., 2009). Glycoproteins from these plants carry almost exclusively Man9GlcNAc2 oligomannosidic N-glycans, but the molecular targets and underlying processes that are defective in the absence of mannose trimming are unknown (Strasser, 2014).
Recently, a study reported that mannose trimming reactions catalyzed by MNS1 and MNS2 play a crucial role for the salt stress tolerance of Arabidopsis (Liu et al., 2018). Under salt stress conditions, the stability of the heavily glycosylated endo-β1,4-glucanase KORRIGAN1 (RSW2) is compromised by pharmacologically inhibition of α-mannosidases or in the mns12 double mutant. Arabidopsis RSW2 is involved in cellulose biosynthesis and has been linked to salt stress sensitivity in a previous study (Kang et al., 2008). Moreover, the genetic interaction between a temperature-sensitive rsw2 allele and N-glycan processing mutants is well established (Kang et al., 2008; von Schaewen et al., 2008; Liebminger et al., 2009, 2010; Rips et al., 2014). In summary, these studies provide evidence for a link between N-glycan maturation, cellulose biosynthesis and the salt stress response of roots.
Despite their requirement for the full assembly of the lipid-linked oligosaccharide precursor, deficiency of ALG3(Henquet et al., 2008; Kajiura et al., 2010), ALG12 (Hong et al., 2009), or ALG9 (Hong et al., 2012) is tolerated by Arabidopsis and does not cause an obvious growth or developmental phenotype. Glycoproteins from these plants display only mild underglycosylation indicating that the mannose residues on the B- and C-branches are less critical for the overall N-glycosylation efficiency. Interestingly, an Arabidopsis mutant lacking a functional ALG9 partially suppresses the salt sensitivity of MNS1/MNS2-deficient plants (Liu et al., 2018). Whether the ALG3, ALG9, or ALG12 disruption can rescue the root growth defect of MNS-deficient plants under normal growth conditions has not been examined yet. Here, we investigated whether plants with defined defects in the biosynthesis of the B- and C-branches of the lipid-linked oligosaccharide precursor impact the vegetative growth phenotype of the Arabidopsis mns123 mutant under non-stress conditions.
Materials and Methods
Plant Material
The mns mutants (mns3 single, mns12 double, and mns123 triple mutant) were available from a previous study (Liebminger et al., 2009). The os9 single mutant has been described previously (Hüttner et al., 2012). Homozygous alg3 (SALK_064006) (Hüttner et al., 2014), alg9 (GABI_831D07), and alg12 (FLAG_310A12) (Hüttner et al., 2012) T-DNA insertion lines were identified by PCR from genomic DNA and crossed with mns123 to generate the respective quadruple mutants. The gntI (SALK_073560, also called cgl1-T) T-DNA insertion mutant (Frank et al., 2008) was crossed with alg3 and mns3, respectively, to obtain the gntI alg3 and gntI mns3 double mutants. The os9 single mutant was crossed with mns123 and mns123 alg3 to obtain the quadruple mutant mns123 os9 and the quintuple mutant mns123 alg3 os9. Arabidopsis thaliana wild-type and mutants were grown under long-day conditions (16-h-light/8-h-dark photoperiod) at 22°C. For mannosidase inhibitor treatments, seeds were directly germinated on 0.5 × Murashige and Skoog (MS) medium containing 2% sucrose and 20 μM kifunensine (Santa Cruz Biotechnology). For root length measurements, the different mutants were grown for 7 days on 0.5 × MS containing 1% sucrose. The seedlings were scanned and the primary root length was measured using Image J software. Four biological replicates were used to determine the root length. For quantification of rosette area and diameter (maximum distance between two points on the rosette boundary), pictures were taken from 23-day-old soil-grown plants and analyzed using Image J (Schneider et al., 2012).
Immunoblotting and N-Glycan Analysis
Proteins were extracted from 7-day-old Arabidopsis seedlings with Laemmli sample buffer. The extracts were separated by SDS-PAGE and analyzed by immunoblotting with anti-horseradish peroxidase (HRP) antibodies to detect complex N-glycans carrying β1,2-xylose and core α1,3-fucose (Liebminger et al., 2009). The myrosinase TGG1 and the protein disulfide isomerase PDI5 were monitored using custom-made polyclonal antibodies as described in detail previously (Veit et al., 2015). GFP-tagged proteins were detected using an anti-GFP antibody (Roche). Endo H (New England Biolabs) digestions were done as described in detail recently from proteins of 12-day-old seedlings (Hüttner et al., 2012). N-linked glycan purification and matrix-assisted laser desorption ionization (MALDI) mass spectrometry (MS) were carried out as described (Liebminger et al., 2009).
Cloning and Transformation of mns123
The constructs for complementation of the root growth phenotype of Arabidopsis mns123 were generated in the following way. The cDNA region coding for the MNS1 (At1g51590) catalytic domain (CD: amino acids 89-560) was synthesized by GeneArt Gene Synthesis (Thermo Fisher Scientific) to remove an internal XbaI site and the BamHI/BglII digested fragment was cloned into the BamHI site of p47 (Hüttner et al., 2014) to generate p47-MNS1CD. The MNS1 promoter region was amplified by PCR from genomic DNA with 5′-TATAGGTACCGGTTGCTTTTCATCAATCTACCTAA-3′ and 5′-TATATCTAGATTCTCAACCCACTCAACAAAAAC-3′, KpnI/XbaI digested and cloned into p47-MNS1CD to generate p87-MNS1:MNS1CD. Next, the cDNA coding for the MNS1-CTS region (amino acids 1-88, CTS stands for the N-terminal cytoplasmic-transmembrane domain and stem region) (Liebminger et al., 2009) or the ST-CTS region (amino acids 1-52) (Boevink et al., 1998) were inserted into XbaI/BamHI sites to generate p87-MNS1:MNS1CTS-MNS1CD and p87-MNS1:STCTS-MNS1CD, respectively. In the p87 vector, MNS1 is expressed from its endogenous promoter and carries a C-terminal GFP tag. Transgenic Arabidopsis were subsequently generated by floral dipping of mns123 and selection on 0.5 × MS medium supplemented with hygromycin. The presence of the transgene was verified by PCR from genomic DNA using MNS1 and CTS-region specific primers.
To express MNS1 fused to mRFP in N. benthamiana, the full-length Arabidopsis MNS1 coding sequence was cloned into p48 (Hüttner et al., 2014) to generate p48-MNS1 following the same cloning strategy as outlined previously for p20-MNS1 (Schoberer et al., 2014). For expression of the ST-CTS-MNS1-catalytic domain fusion protein (ST-MNS1) under the control of the ubiquitin 10 promoter (p47 vector), the ST-CTS region was cloned into the XbaI/BamHI site of p47-MNS1CD resulting in the expression vector p47-ST-MNS1.
Confocal Microscopy
Transient expression in Nicotiana benthamiana leaf epidermal cells was done by agrobacterium-mediated infiltration of leaves (Schoberer et al., 2009). For co-expression experiments, agrobacteria were diluted to an OD600 of 0.1 for p48-MNS1 (RFP-tagged full-length MNS1 expressed under the control of the ubiquitin 10 promoter) and pVKH18-En6:STmRFP (RFP-tagged ST-CTS region expressed under the control of the CaMV35S promoter) (Renna et al., 2005). An OD600 of 0.2 was used for p20-MNS1 (GFP-tagged full-length MNS1 expressed under the control of the CaMV35S promoter) and p47-ST-MNS1 (GFP-tagged fusion protein expressed under the control of the ubiquitin 10 promoter). Sampling and imaging of fluorescent proteins was performed 2 days after infiltration using a Leica TCS SP5 confocal microscope as described in detail previously (Schoberer et al., 2014). Postacquisition image processing was performed in Adobe Photoshop CS and Image J. For co-localization analysis, infiltrated leaf disks were (prior to image acquisition) treated for 30–45 min with the actin-depolymerizing agent latrunculin B (Merck Millipore) at a concentration of 25 μM to inhibit Golgi movement. Coefficients were calculated from selected Golgi stacks using Image J (Schneider et al., 2012) and the plugin JACoP (Bolte and Cordelières, 2006).
Results
N-Glycans Are Altered in alg Mutants With Defects in Mannose Trimming
To examine whether ALG-deficient mutants such as alg3, alg9, and alg12 affect the mns123 phenotype we generated mns123 alg3, mns123 alg9, and mns123 alg12 quadruple mutants. Based on the known biosynthetic and processing reactions of these enzymes, it is predicted that all of the Arabidopsis mutants carry exclusively oligomannosidic N-glycans with a distinct structural composition (Supplementary Figure 1). In addition, we included the gntI T-DNA insertion mutant (Frank et al., 2008) and crosses of gntI with mns3 (gntI mns3 double mutant) as well as gntI crossed with alg3 (gntI alg3 double mutant) in our analysis because they produce distinct oligomannosidic N-glycans (von Schaewen et al., 1993; Henquet et al., 2008; Liebminger et al., 2009). To verify the absence of complex N-glycans in all the mutants, we extracted proteins from seedlings as well as rosette leaves and carried out immunoblotting with antibodies against β1,2-xylose and core α1,3-fucose residues that are characteristic for complex N-glycans (Strasser, 2014). As expected, none of the mutants displayed a signal showing the complete absence of complex N-glycans (Figure 1C and Supplementary Figure 2). Moreover, immunoblot analysis of the myrosinase TGG1 which is heavily glycosylated and carries mainly oligomannosidic N-glycans (Liebminger et al., 2012) or a protein disulfide isomerase (PDI) carrying two N-glycans revealed differences in mobility compared to the same proteins from wild-type (Figure 1D). We digested the protein extracts from the mutants displaying the fastest migrating TGG1 with endoglycosidase H (Endo H) to remove the oligomannosidic glycans and repeated the immunoblot. Upon digestion, the fastest migrating TGG1 was observed for mns123 alg9, mns123, and gntI. This band likely represents TGG1 with all oligomannosidic N-glycans removed (Figure 1E). Wild-type TGG1 appeared as a more diffuse band which can be explained by the presence of small amounts of Endo H-resistant complex-type N-glycans that lead to a slightly higher molecular weight and reduced mobility on immunoblots (Liebminger et al., 2012). Endo H digested TGG1 from mns123 alg3 and gntI alg3 displayed no clear shift in mobility compared to the undigested proteins indicating the presence of altered oligomannosidic N-glycans (Figure 1E).
To determine the N-glycan structures in the mutants more precisely, we harvested 500 mg leaves from 5-week-old soil-grown plants, purified the N-glycans and analyzed them by MALDI MS. In accordance with immunoblot data, the major peak corresponds to a single mannosidic N-glycan in all mutants (Figure 2). Whereas in wild-type Col-0, in alg3 (Henquet et al., 2008), alg12, and alg9 (Supplementary Figure 3) single mutants the majority of N-glycans are of the complex or paucimannosidic-type, the different mutants displayed Man9GlcNAc2 (mns123), Man8GlcNAc2 (mns12), Man7GlcNAc2 (mns123 alg12), Man6GlcNAc2 (mns123 alg9), Man5GlcNAc2 (mns123 alg3), Man6GlcNAc2 (gntI mns3), Man5GlcNAc2 (gntI), and Man3GlcNAc2 (gntI alg3) structures. Thus, the MALDI MS data are consistent with the immunoblot data and confirm that the biosynthesis and processing defects cause the proposed alteration of N-glycan structures as outlined in Supplementary Figure 1.
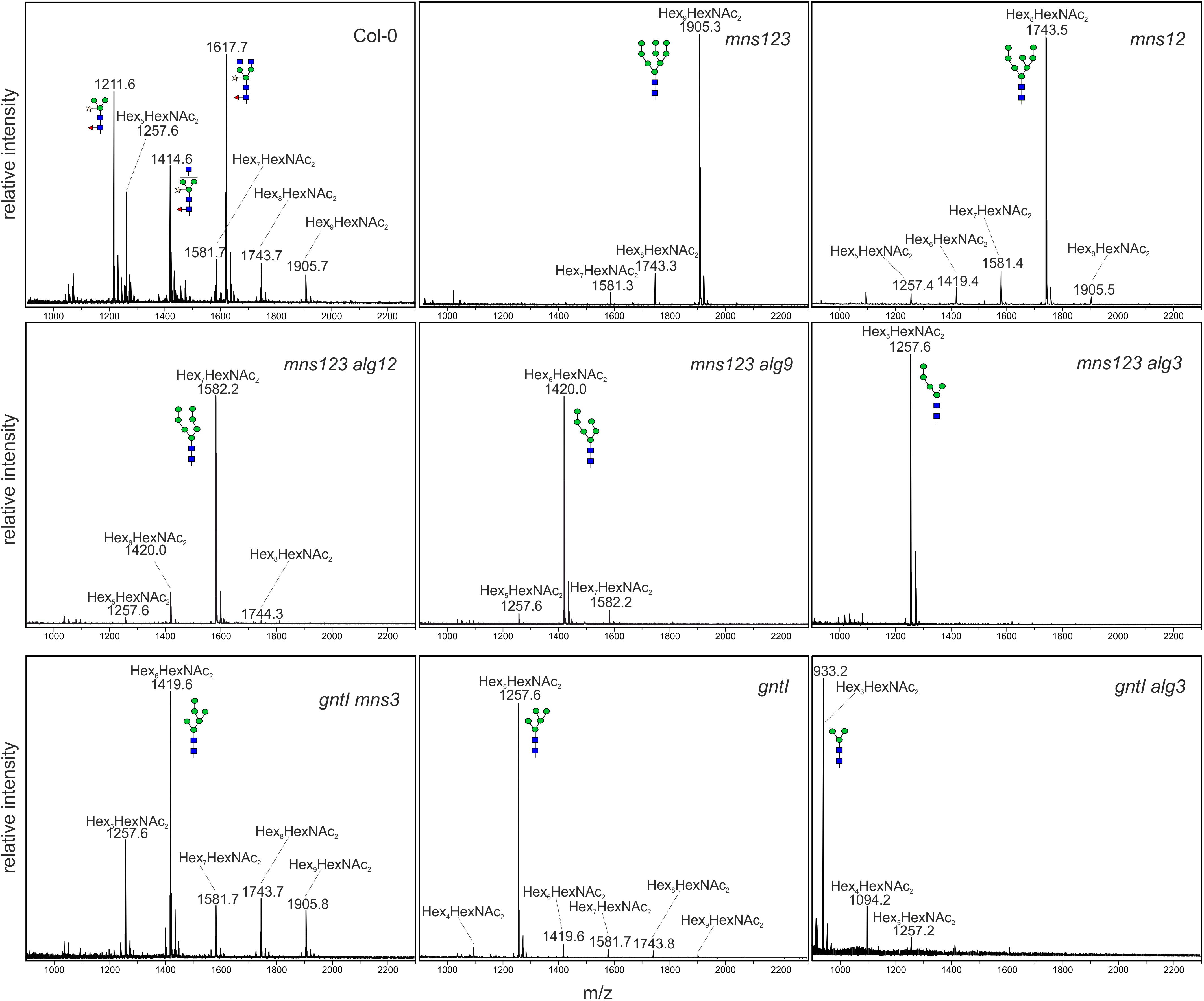
Figure 2. MALDI MS analysis of N-glycans isolated from rosette leaves of 5-week-old wild-type (Col-0) or different Arabidopsis mutants.
Deficiency of ALGs Rescues the Root Growth Defect of mns123
To investigate the impact of altered N-glycan structures and impaired mannose trimming on plant growth, we compared the primary root length of all characterized mutants (Figures 3A,B and Supplementary Figure 4). Intriguingly, the previously described severe root growth defect (Liebminger et al., 2009) was only found for mns123 containing unprocessed Man9GlcNAc2 structures. The mns123 alg12, mns123 alg9, and mns123 alg3 quadruple mutants displayed a wild-type like root phenotype indicating that the absence of mannose residues at the B- or C-branches of the N-glycans rescues the mns123 root growth phenotype. The primary root of the gntI alg3 double mutant was also significantly shorter compared to wild-type but clearly less affected than mns123. A suppression of the mns123 phenotype was also seen when mns123 alg3 plants were grown on soil (Figure 3C) or on MS-medium in the dark (Supplementary Figure 4). The rosette area and the maximum rosette diameter of mns123 alg3 were smaller than wild-type, but significantly larger than mns123 (Figure 3D). A suppression of the mns123 phenotype was detected for mns123 alg12 and mns123 alg9 (data not shown).
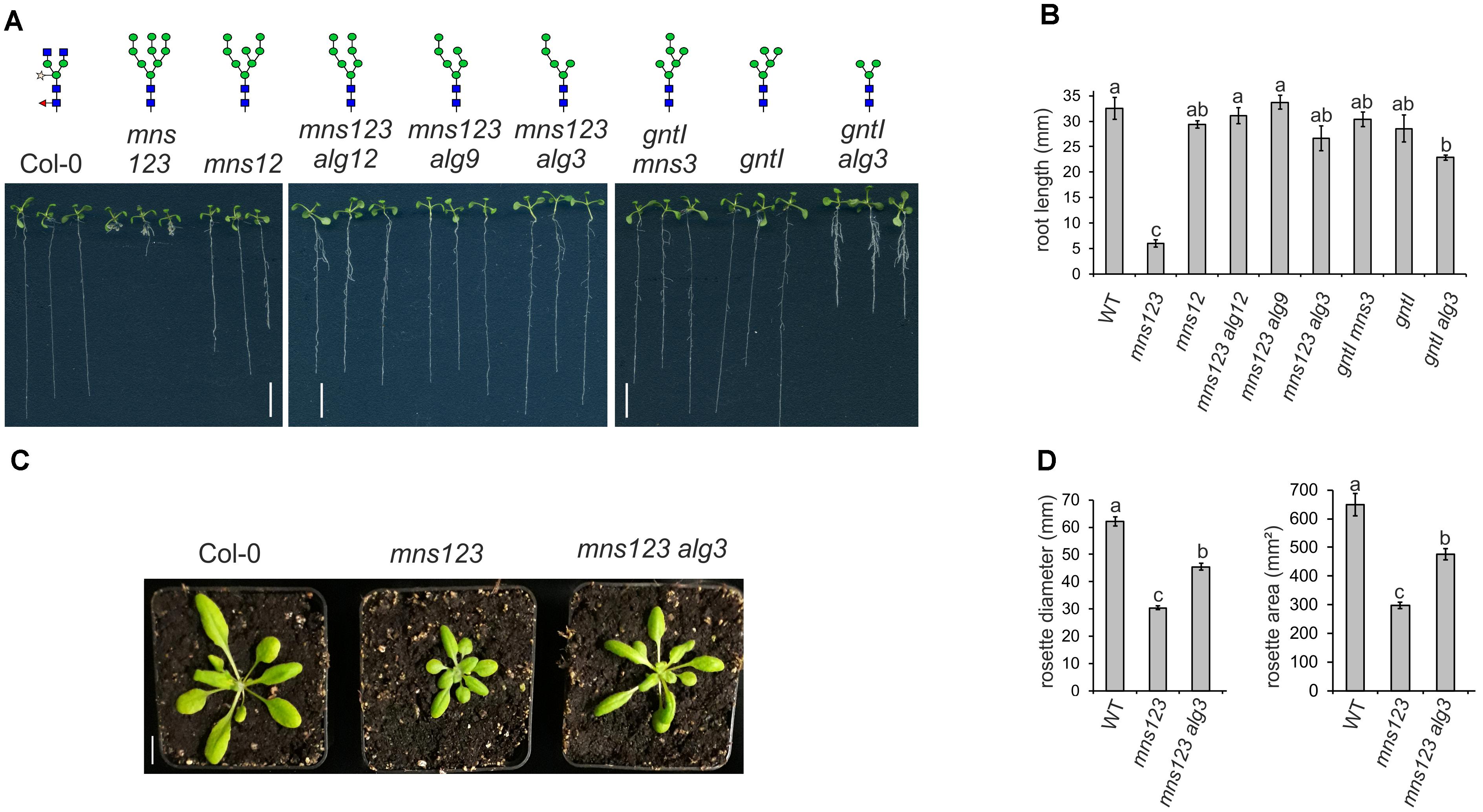
Figure 3. (A) The root growth phenotype of 9-day-old Arabidopsis seedlings with defects in lipid-linked oligosaccharide precursor biosynthesis and/or N-glycan processing. Seedlings were grown vertically on 0.5 × MS containing 2% sucrose. The major N-glycan structures in these lines are indicated as illustrations. Scale bar = 1 cm. (B) The primary root length of WT and the different mutants when grown for seven days on 0.5 × MS containing 1% sucrose are shown. Data represent mean values ± standard error. Data were analyzed using one-way ANOVA with Tukey’s post hoc-test (four biological replicates, 25–110 seedlings/each). Different letters indicate significant differences among different genotypes (P < 0.05). (C) Phenotype of 4-week-old soil grown Arabidopsis Col-0, mns123, and mns123 alg3. Scale bar = 1 cm. (D) Quantification of the maximum rosette diameter and the rosette area of 23-day-old plants. Data represent mean values ± standard error (n ≥ 27 plants). Data were analyzed using one-way ANOVA with Tukey’s post hoc-test. Different letters indicate significant differences among different genotypes (P < 0.01).
Next, we assessed the response of alg3, alg9, and alg12 seedlings to kifunensine, a specific class I α-mannosidase inhibitor (Elbein et al., 1990). In a previous study, we have shown that kifunensine blocks MNS1 to MNS3-mediated mannose trimming and leads to a root growth defect of wild-type seedlings that is reminiscent of the mns123 phenotype (Liebminger et al., 2009). In contrast to wild-type, the root growth of the three alg single mutants appeared insensitive to pharmacological inhibition of α-mannosidases (Figure 4A). Likewise, the quadruple mutants displayed long roots in the presence of kifunensine. Taken together, these data are consistent with the observed suppression of the mns123 phenotype in the ALG-deficient mutants.
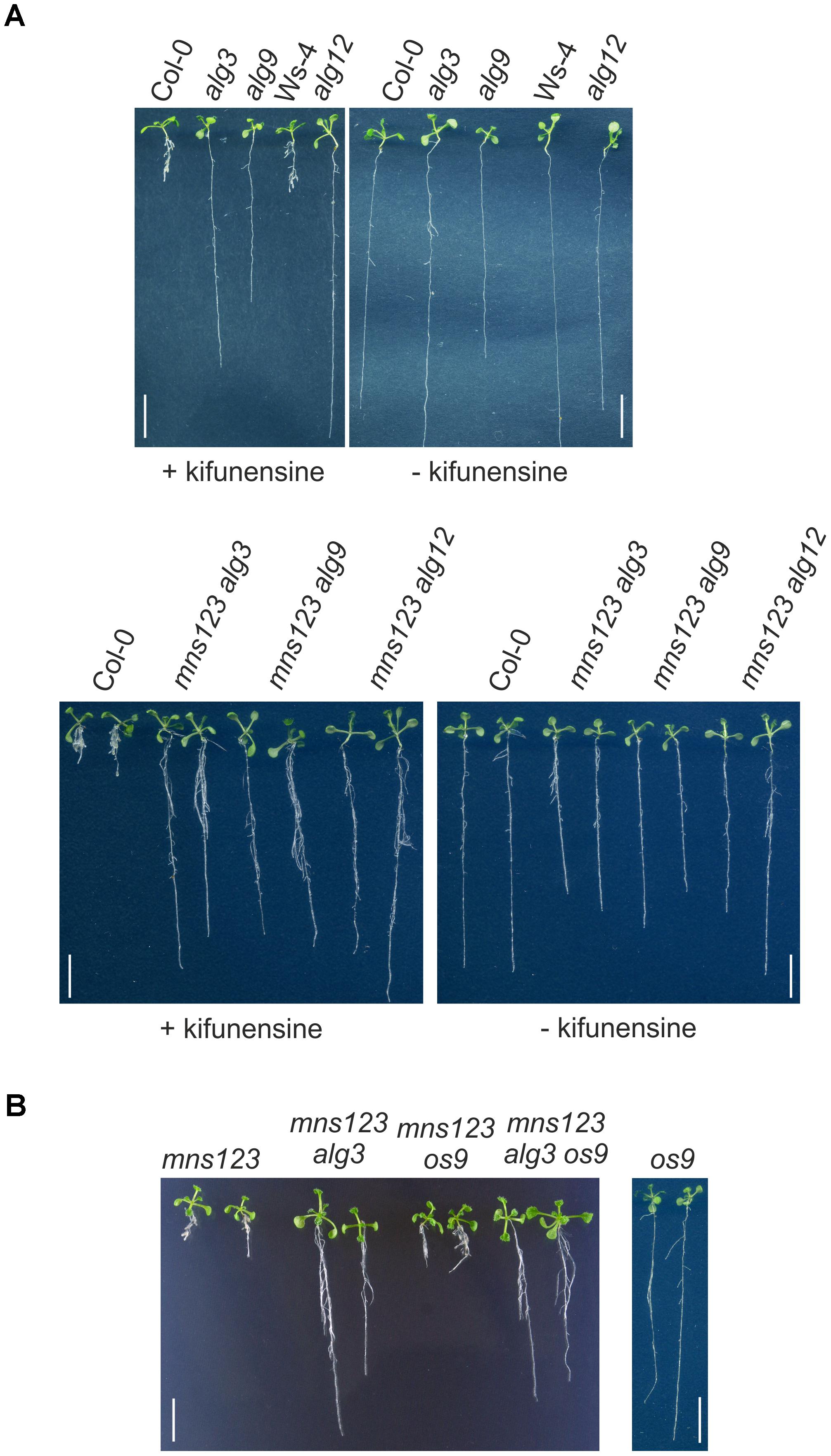
Figure 4. (A) Root phenotype of Arabidopsis Col-0, Ws-4 (parental line for alg12), different alg single mutants, and the mns123 alg quadruple mutants in the presence of kifunensine. Seedlings were grown for 10 days on 0.5 × MS containing 2% sucrose in the presence or absence of 20 μM kifunensine. Scale bar = 1 cm. (B) Root phenotype of 14-day-old Arabidopsis seedlings grown on 0.5 × MS supplemented with 1% sucrose. Scale bar = 1 cm.
Suppression of the Root Growth Defect Is Uncoupled From Glycan-Dependent ERAD
Kifunensine is also used as specific inhibitor for ER-associated degradation (ERAD) of misfolded glycoproteins (Hüttner et al., 2012) and alg9 as well as alg12 have been identified as specific suppressors of ERAD in Arabidopsis (Hong et al., 2009, 2012). Therefore, we examined whether the observed rescue of the mns123 growth phenotype is caused by an inhibition of the ERAD pathway. To this end, we crossed mns123 with the os9 mutant lacking a functional variant of the carbohydrate binding protein OS9 (Hüttner et al., 2012). Together with other proteins, OS9 is part of the HRD1 complex that selects aberrant glycoproteins to glycan-dependent ERAD. The mns123 os9 quadruple mutant displayed the same root growth defect like mns123 and the mns123 alg3 os9 quintuple mutant phenocopied mns123 alg3 showing that the observed phenotypic suppression is independent of a functional ERAD pathway (Figure 4B).
Golgi-Mediated Mannose Trimming Rescues the Root Growth Defect
To investigate whether the observed mns123 root growth phenotype is caused by the inability to process N-glycans in the Golgi apparatus, we expressed MNS1-GFP under its own promoter with its endogenous cis/medial-Golgi targeting and retention signal (MNS1-CTS region) (Liebminger et al., 2009) in the mns123 mutant. MNS1-GFP expression restored the formation of complex N-glycans and normal root growth showing that this transgene is functional (Figures 5A,B and Supplementary Figure 5).
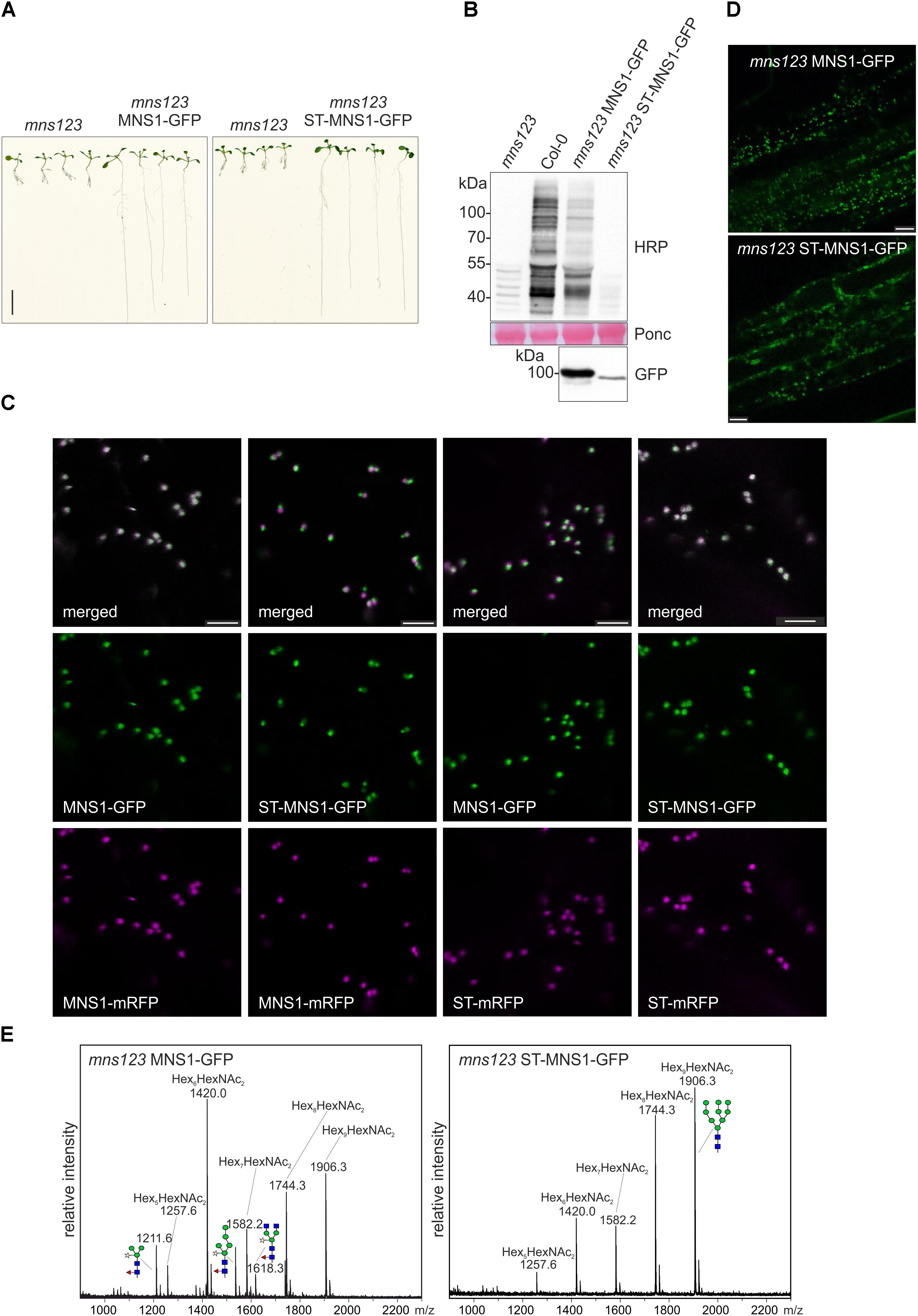
Figure 5. (A) Seedlings grown on 0.5 × MS medium containing 1% sucrose for 9 days. Scale bar = 1 cm. (B) Immunoblot analysis using anti-horseradish peroxidase (HRP) antibodies, which recognize β1,2-xylose and core α1,3-fucose residues on N-glycans. Ponceau S (Ponc) staining of the membrane is shown as a loading control and anti-GFP antibody was used to monitor MNS1-GFP and ST-MNS1-GFP expression. (C) Confocal images (2 days after infiltration) of N. benthamiana leaf epidermal cells transiently co-expressing MNS1-mRFP or ST-mRFP with MNS1-GFP or ST-MNS1-GFP. The white color in the merged image shows the co-localization. Scale bare = 5 μm. (D) Confocal images from roots of 10-day-old Arabidopsis mns123 seedlings expressing MNS1-GFP or ST-MNS1-GFP. Scale bar = 10 μm. (E) Leaves from different transgenic lines were pooled and subjected to MALDI MS analysis. The characteristic oligomannosidic N-glycan (Hex5-9HexNAc2) peaks are indicated. Man9GlcNAc2 and different complex/truncated N-glycans with xylose and fucose are shown as illustrations.
Next, we examined whether MNS1 targeting to the trans-Golgi can rescue the root growth phenotype of mns123. To this end, we replaced the N-terminal MNS1-CTS region with the well-known trans-Golgi targeting signal from rat α2,6-sialyltransferase (ST-CTS region) (Boevink et al., 1998). We assumed that the ST-MNS1 fusion protein is targeted to the trans-Golgi because the CTS-region is typically the determinant for sub-Golgi compartmentation of N-glycan processing enzymes in plants (Saint-Jore-Dupas et al., 2006; Schoberer et al., 2013, 2014). To monitor the subcellular localisation we transiently co-expressed ST-MNS1-GFP with marker proteins in N. benthamiana leaf epidermal cells. ST-MNS1-GFP was co-expressed with the cis/medial-Golgi protein MNS1-mRFP (Liebminger et al., 2009; Nebenführ et al., 1999) and the trans-Golgi marker ST-mRFP (Boevink et al., 1998), respectively. Confocal microscopy images of ST-MNS1-GFP and ST-mRFP displayed overlapping signals (Figure 5C) indicating that these proteins reside in the same Golgi cisternae. By contrast, Golgi stacks clearly appeared tricolored when ST-MNS1-GFP was co-expressed with MNS1-GFP indicating distinct intra-Golgi distributions and trans-Golgi accumulation of ST-MNS1-GFP. Co-localization analyses of ST-MNS1-GFP with ST-mRFP corroborated these findings (Table 1). In Arabidopsis seedlings, MNS1-GFP and ST-MNS1-GFP labeled mobile structures resembling Golgi stacks (Figure 5D). Compared to MNS1-GFP, the fluorescence signal was much lower for ST-MNS1-GFP expressing plants and ST-MNS1-GFP protein levels were reduced (Figure 5B). The lower amounts of ST-MNS1-GFP may be explained by the secretion of excess ST-fusion protein from the Golgi (Runions et al., 2006). Nonetheless, ST-MNS1-GFP expression resulted in suppression of the mns123 root growth defect (Figure 5A). Immunoblot and MALDI MS analyses revealed the absence of complex N-glycans in ST-MNS1-GFP expressing mns123 (Figures 5B,E). However, a considerable amount of Man9GlcNAc2 N-glycans was processed to Man8GlcNAc2 and other oligomannosidic structures. In summary, these data indicate that removal of mannose residues in a late Golgi compartment is sufficient to rescue the root growth defect.
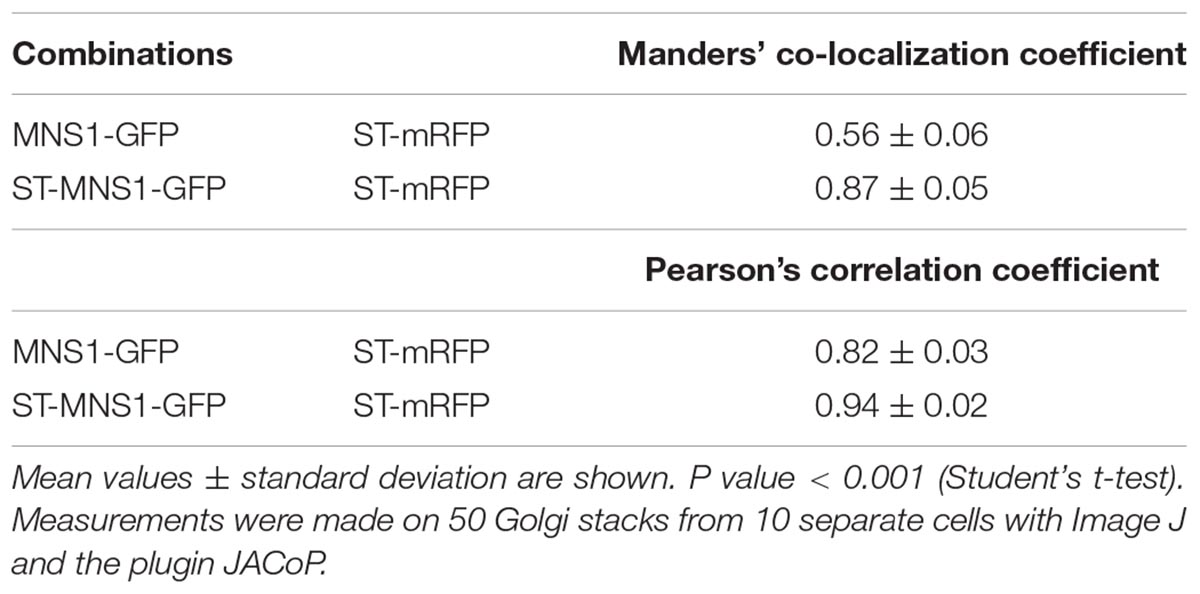
Table 1. Co-localization of MNS1-GFP and ST-MNS1-GFP with the trans-Golgi marker ST-mRFP in N. benthamiana leaf epidermal cells.
Discussion
Impaired mannose trimming from oligomannosidic N-glycans results in a severe root phenotype and subsequent growth defects of aerial rosettes (Liebminger et al., 2009). The underlying process(es) and affected glycoproteins have not been discovered yet. Plants contain an entire set of ALG glycosyltransferases to synthesize the lipid-linked oligosaccharide precursor composed of 14 sugars. The previously described alg10 knockout plants display a leaf growth defect (Farid et al., 2011) and lew3 (defect in the Arabidopsis ALG11 gene) null mutants are embryo lethal (Zhang et al., 2009). By contrast, ALG3, ALG9, and ALG12-deficient Arabidopsis display a wild-type like growth despite the fact that they have altered oligomannosidic N-glycans (Henquet et al., 2008; Hong et al., 2009, 2012). Even under salt/osmotic stress conditions, the root growth of alg3 is comparable to wild-type (Kajiura et al., 2010) suggesting that the absence of mannose residues on the B- and C-branches of the assembled oligosaccharide is well tolerated by the plants and does not severely interfere with developmental processes. In agreement with these findings, our data show that ALG3, ALG9, and ALG12 deficiency rescue the growth phenotype of mns123. The removal of mannose residues from the B- and C-branches is not only crucial for salt stress tolerance as shown recently (Liu et al., 2018), but also for normal growth. Liu and colleagues reported that RSW2 stability is affected under salt stress conditions when mannose trimming is blocked on the C-branch (Liu et al., 2018). The authors proposed that the unprocessed α1,2-mannose residue on the C-branch is recognized by an as yet unknown carbohydrate binding protein which may divert glycoproteins like RSW2 for degradation under salt stress conditions. We did not examine the fate of RSW2 under our growth conditions because previous studies have shown that N-glycan processing does not have a direct effect on RSW2 function (Liebminger et al., 2013; Rips et al., 2014). Moreover, the mns12 double mutant with the unprocessed C-branch displays a much less severe root phenotype compared to mns123. Based on these data, it is likely that the salt stress sensitivity and the root phenotype observed under normal growth conditions involve different glycoproteins and processes. Whether specific lectin-like receptors recognize glycoproteins with unprocessed terminal mannose residues at the B- and/or C-branches remains to be shown in future studies. Our data suggest that such lectins would bind with the highest affinity to Man9GlcNAc2 while N-glycans lacking a terminal α1,2-mannose residue on either the B (e.g., mns12) or C-branch (e.g., mns123 alg12) display reduced interaction.
In the ER, a glycan-dependent ERAD pathway removes misfolded glycoproteins. This process involves the generation of a conserved glycan signal with an exposed terminal α1,6-mannose residue on the C-branch that is subsequently recognized by the lectin OS9. The binding of OS9 selects substrates for specific degradation via the SEL1L-HRD1 complex (Clerc et al., 2009; Su et al., 2011; Hong et al., 2012; Hüttner et al., 2014). OS9 is a mannose 6-phosphate receptor homology (MRH) domain-containing protein that resides in the ER in plants. Here, we provide genetic evidence that OS9 and glycan-dependent ERAD can be uncoupled from the root growth defect. We generated a chimeric ST-MNS1 variant that was targeted to the trans-Golgi in N. benthamiana leaf epidermal cells and rescued the mns123 root growth defect. Although not directly confirmed by co-localization in seedlings, we propose that the ST-MNS1 variant is located in the trans-Golgi in Arabidopsis as shown previously for full-length ST (Wee et al., 1998). The late Golgi-targeting in Arabidopsis is supported by the absence of complex N-glycans in ST-MNS1-GFP expressing plants. Importantly, ST-MNS1-GFP expression resulted in the cleavage of mannose residues from oligomannosidic N-glycans and the suppression of the mns123 root phenotype indicating that a late Golgi or post-Golgi event is abolished in the absence of mannose trimming. Such an event could be a specific recognition by a mannose-binding lectin that directs glycoproteins with unprocessed oligomannosidic N-glycans to a degradation pathway. Alternatively, a biologically relevant protein–protein interaction could be affected in the mns123 mutant due to the presence of Man9GlcNAc2 N-glycans leading to the observed growth phenotype.
In mammals, MRH domain-containing lectins are found in other parts of the secretory pathway including the Golgi. Binding of MRH-domain containing receptors to mannose-6-phosphate from N-glycans of cargo glycoproteins leads to their transport from the trans-Golgi network (TGN) to the lysosome (Castonguay et al., 2011). MRH domain-containing receptors implicated in targeting of lysosomal/vacuolar enzymes have been described in Drosophila and yeast. Plants, however, lack homologs of these receptors (de Marcos Lousa and Denecke, 2016). Besides OS9, the only known MRH domain-containing protein in plants is the β-subunit from α-glucosidase II that resides in the ER and modulates the N-glycan processing activity of the α-glucosidase II α-subunit (Lu et al., 2009; Olson et al., 2013). Other lectin-types such as mammalian ERGIC-53 and VIP36 are known to bind to oligomannosidic glycans of cargo glycoproteins and are involved in their transport from the ER to the ER-Golgi intermediate compartment (ERGIC) or cis-Golgi (Hauri et al., 2000). The Arabidopsis genome does not contain homologs of these proteins and similar cargo receptors involved in ER to Golgi transport have not been described in plants. On the other hand, Arabidopsis has numerous uncharacterized lectin domain containing proteins like lectin receptor kinases that could be involved in binding to specific oligomannosidic N-glycans (Eggermont et al., 2017; Teixeira et al., 2018). For example, Galanthus nivalis agglutinin (GNA) binds preferentially to terminal α1,3-linked mannose residues and to a lesser extend also to α1,6-linked mannose containing structures (Shibuya et al., 1988). Arabidopsis contains 49 putative lectin genes coding for proteins with a GNA domain and almost all of them have either a signal peptide sequence or transmembrane domain (Eggermont et al., 2017) which makes them potential candidates for lectins binding to oligomannosidic N-glycans in the secretory pathway.
In addition to regulation of lectin binding events, the presence or absence of certain mannose residues on N-glycans of secretory proteins may directly affect the protein conformation and consequently the interaction with other proteins. Plant soluble vacuolar proteins are typically sorted by specific vacuolar sorting receptors (VSRs) that recognize amino acid motifs from cargo proteins. Arabidopsis VSR1 is glycosylated with complex N-glycans and N-glycosylation stabilizes its ligand binding conformation (Shen et al., 2014). Moreover, there is evidence that VSRs can bind their cargo already in the ER where the VSR N-glycans would still be oligomannosidic (Künzl et al., 2016). It is therefore tempting to speculate that the cargo-binding affinity of glycosylated VSRs such as VSR1 is not only influenced by N-glycosylation but also by a distinct N-glycan structure. In this scenario, unprocessed oligomannosidic N-glycans could provide stronger cargo binding than partially processed oligomannosidic or complex N-glycans. The stepwise ER to trans-Golgi mediated processing of N-glycans could represent an elegant mechanism to alter cargo affinity and enable the controlled cargo release on their route to the vacuole. Whether the VSR N-glycan composition has indeed an impact on cargo binding and how such a glycan-mediated process can be integrated with our findings and with recent data showing that recycling VSRs bind cargo in the cis-Golgi (Früholz et al., 2018) remains to be shown in future studies.
Author Contributions
CV, JK, FA, and RS have made a substantial and intellectual contribution to the work and approved it for publication.
Funding
This work was supported by a grant from the Austrian Science Fund (FWF): P28218-B22.
Conflict of Interest Statement
The authors declare that the research was conducted in the absence of any commercial or financial relationships that could be construed as a potential conflict of interest.
Acknowledgments
We thank Akhlaq Farid, Silvia Hüttner, Eva Liebminger, Jennifer Schoberer, and Ulrike Vavra for help with the analysis and screening of Arabidopsis mutants and Karin Polacsek for glycan purification and MALDI MS analysis. We thank the BOKU-VIBT Imaging Center for access and expertise.
Supplementary Material
The Supplementary Material for this article can be found online at: https://www.frontiersin.org/articles/10.3389/fpls.2018.01807/full#supplementary-material
References
Aebi, M. (2013). N-linked protein glycosylation in the ER. Biochim. Biophys. Acta 1833, 2430–2437. doi: 10.1016/j.bbamcr.2013.04.001
Boevink, P., Oparka, K., Santa Cruz, S., Martin, B., Betteridge, A., and Hawes, C. (1998). Stacks on tracks: the plant Golgi apparatus traffics on an actin/ER network. Plant J. 15, 441–447. doi: 10.1046/j.1365-313X.1998.00208.x
Boisson, M., Gomord, V., Audran, C., Berger, N., Dubreucq, B., Granier, F., et al. (2001). Arabidopsis glucosidase I mutants reveal a critical role of N-glycan trimming in seed development. EMBO J. 20, 1010–1019. doi: 10.1093/emboj/20.5.1010
Bolte, S., and Cordelières, F. P. (2006). A guided tour into subcellular colocalization analysis in light microscopy. J. Microsc. 224, 213–232. doi: 10.1111/j.1365-2818.2006.01706.x
Castonguay, A. C., Olson, L. J., and Dahms, N. M. (2011). Mannose 6-phosphate receptor homology (MRH) domain-containing lectins in the secretory pathway. Biochim. Biophys. Acta 1810, 815–826. doi: 10.1016/j.bbagen.2011.06.016
Clerc, S., Hirsch, C., Oggier, D., Deprez, P., Jakob, C., Sommer, T., et al. (2009). Htm1 protein generates the N-glycan signal for glycoprotein degradation in the endoplasmic reticulum. J. Cell Biol. 184, 159–172. doi: 10.1083/jcb.200809198
de Marcos Lousa, C., and Denecke, J. (2016). Lysosomal and vacuolar sorting: not so different after all! Biochem. Soc. Trans. 44, 891–897. doi: 10.1042/BST20160050
Eggermont, L., Verstraeten, B., and Van Damme, E. J. M. (2017). Genome-wide screening for lectin motifs in Arabidopsis thaliana. Plant Genome 10. doi: 10.3835/plantgenome2017.02.0010
Elbein, A. D., Tropea, J. E., Mitchell, M., and Kaushal, G. P. (1990). Kifunensine, a potent inhibitor of the glycoprotein processing mannosidase I. J. Biol. Chem. 265, 15599–15605.
Fanata, W. I., Lee, K. H., Son, B. H., Yoo, J. Y., Harmoko, R., Ko, K. S., et al. (2013). N-glycan maturation is crucial for cytokinin-mediated development and cellulose synthesis in Oryza sativa. Plant J. 73, 966–979. doi: 10.1111/tpj.12087
Farid, A., Pabst, M., Schoberer, J., Altmann, F., Glössl, J., and Strasser, R. (2011). Arabidopsis thaliana alpha1,2-glucosyltransferase (ALG10) is required for efficient N-glycosylation and leaf growth. Plant J. 68, 314–325. doi: 10.1111/j.1365-313X.2011.04688.x
Frank, J., Kaulfürst-Soboll, H., Rips, S., Koiwa, H., and von Schaewen, A. (2008). Comparative analyses of Arabidopsis complex glycan1 mutants and genetic interaction with staurosporin and temperature sensitive3a. Plant Physiol. 148, 1354–1367. doi: 10.1104/pp.108.127027
Früholz, S., Fäßler, F., Kolukisaoglu,Ü, and Pimpl, P. (2018). Nanobody-triggered lockdown of VSRs reveals ligand reloading in the Golgi. Nat. Commun. 9:643. doi: 10.1038/s41467-018-02909-6
Gillmor, C., Poindexter, P., Lorieau, J., Palcic, M., and Somerville, C. (2002). Alpha-glucosidase I is required for cellulose biosynthesis and morphogenesis in Arabidopsis. J. Cell Biol. 156, 1003–1013. doi: 10.1083/jcb.200111093
Harmoko, R., Yoo, J. Y., Ko, K. S., Ramasamy, N. K., Hwang, B. Y., Lee, E. J., et al. (2016). N-glycan containing a core α1,3-fucose residue is required for basipetal auxin transport and gravitropic response in rice (Oryza sativa). New Phytol. 212, 108–122. doi: 10.1111/nph.14031
Hauri, H. P., Kappeler, F., Andersson, H., and Appenzeller, C. (2000). ERGIC-53 and traffic in the secretory pathway. J. Cell Sci. 113, 587–596.
Henquet, M., Lehle, L., Schreuder, M., Rouwendal, G., Molthoff, J., Helsper, J., et al. (2008). Identification of the gene encoding the {alpha}1,3-Mannosyltransferase (ALG3) in Arabidopsis and characterization of downstream N-Glycan processing. Plant Cell 20, 1652–1664. doi: 10.1105/tpc.108.060731
Hong, Z., Jin, H., Fitchette, A., Xia, Y., Monk, A., Faye, L., et al. (2009). Mutations of an alpha1,6 mannosyltransferase inhibit endoplasmic reticulum-associated degradation of defective brassinosteroid receptors in Arabidopsis. Plant Cell 21, 3792–3802. doi: 10.1105/tpc.109.070284
Hong, Z., Kajiura, H., Su, W., Jin, H., Kimura, A., Fujiyama, K., et al. (2012). Evolutionarily conserved glycan signal to degrade aberrant brassinosteroid receptors in Arabidopsis. Proc. Natl. Acad. Sci. U.S.A. 109, 11437–11442. doi: 10.1073/pnas.1119173109
Hüttner, S., Veit, C., Schoberer, J., Grass, J., and Strasser, R. (2012). Unraveling the function of Arabidopsis thaliana OS9 in the endoplasmic reticulum-associated degradation of glycoproteins. Plant Mol. Biol. 79, 21–33. doi: 10.1007/s11103-012-9891-4
Hüttner, S., Veit, C., Vavra, U., Schoberer, J., Liebminger, E., Maresch, D., et al. (2014). Arabidopsis class I α-mannosidases MNS4 and MNS5 are involved in endoplasmic reticulum-associated degradation of misfolded glycoproteins. Plant Cell 26, 1712–1728. doi: 10.1105/tpc.114.123216
Jeong, I. S., Lee, S., Bonkhofer, F., Tolley, J., Fukudome, A., Nagashima, Y., et al. (2018). Purification and characterization of Arabidopsis thaliana oligosaccharyltransferase complexes from the native host: a protein super-expression system for structural studies. Plant J. 94, 131–145. doi: 10.1111/tpj.13847
Kajiura, H., Seki, T., and Fujiyama, K. (2010). Arabidopsis thaliana ALG3 mutant synthesizes immature oligosaccharides in the ER and accumulates unique N-glycans. Glycobiology 20, 736–751. doi: 10.1093/glycob/cwq028
Kang, J., Frank, J., Kang, C., Kajiura, H., Vikram, M., Ueda, A., et al. (2008). Salt tolerance of Arabidopsis thaliana requires maturation of N-glycosylated proteins in the Golgi apparatus. Proc. Natl. Acad. Sci. U.S.A. 105, 5933–5938. doi: 10.1073/pnas.0800237105
Koiwa, H., Li, F., McCully, M., Mendoza, I., Koizumi, N., Manabe, Y., et al. (2003). The STT3a subunit isoform of the Arabidopsis oligosaccharyltransferase controls adaptive responses to salt/osmotic stress. Plant Cell 15, 2273–2284. doi: 10.1105/tpc.013862
Künzl, F., Früholz, S., Fäßler, F., Li, B., and Pimpl, P. (2016). Receptor-mediated sorting of soluble vacuolar proteins ends at the trans-Golgi network/early endosome. Nat. Plants 2:16017. doi: 10.1038/nplants.2016.17
Lerouxel, O., Mouille, G., Andème-Onzighi, C., Bruyant, M., Séveno, M., Loutelier-Bourhis, C., et al. (2005). Mutants in DEFECTIVE GLYCOSYLATION, an Arabidopsis homolog of an oligosaccharyltransferase complex subunit, show protein underglycosylation and defects in cell differentiation and growth. Plant J. 42, 455–468. doi: 10.1111/j.1365-313X.2005.02392.x
Liebminger, E., Grass, J., Altmann, F., Mach, L., and Strasser, R. (2013). Characterizing the link between glycosylation state and enzymatic activity of the endo-β1,4-glucanase KORRIGAN1 from Arabidopsis thaliana. J. Biol. Chem. 288, 22270–22280. doi: 10.1074/jbc.M113.475558
Liebminger, E., Grass, J., Jez, J., Neumann, L., Altmann, F., and Strasser, R. (2012). Myrosinases TGG1 and TGG2 from Arabidopsis thaliana contain exclusively oligomannosidic N-glycans. Phytochemistry 84, 24–30. doi: 10.1016/j.phytochem.2012.08.023
Liebminger, E., Hüttner, S., Vavra, U., Fischl, R., Schoberer, J., Grass, J., et al. (2009). Class I alpha-mannosidases are required for N-glycan processing and root development in Arabidopsis thaliana. Plant Cell 21, 3850–3867. doi: 10.1105/tpc.109.072363
Liebminger, E., Veit, C., Mach, L., and Strasser, R. (2010). Mannose trimming reactions in the early stages of the N-glycan processing pathway. Plant Signal. Behav. 5, 476–478. doi: 10.4161/psb.5.4.11423
Liu, C., Niu, G., Zhang, H., Sun, Y., Sun, S., Yu, F., et al. (2018). Trimming of N-glycans by the Golgi-Localized α-1,2-Mannosidases, MNS1 and MNS2, Is crucial for maintaining RSW2 protein abundance during salt stress in Arabidopsis. Mol. Plant 11, 678–690. doi: 10.1016/j.molp.2018.01.006
Lu, X., Tintor, N., Mentzel, T., Kombrink, E., Boller, T., Robatzek, S., et al. (2009). Uncoupling of sustained MAMP receptor signaling from early outputs in an Arabidopsis endoplasmic reticulum glucosidase II allele. Proc. Natl. Acad. Sci. U.S.A. 106, 22522–22527. doi: 10.1073/pnas.0907711106
Nebenführ, A., Gallagher, L., Dunahay, T., Frohlick, J., Mazurkiewicz, A., Meehl, J., et al. (1999). Stop-and-go movements of plant Golgi stacks are mediated by the acto-myosin system. Plant Physiol. 121, 1127–1142. doi: 10.1104/pp.121.4.1127
Olson, L. J., Orsi, R., Alculumbre, S. G., Peterson, F. C., Stigliano, I. D., Parodi, A. J., et al. (2013). Structure of the lectin mannose 6-phosphate receptor homology (MRH) domain of glucosidase II, an enzyme that regulates glycoprotein folding quality control in the endoplasmic reticulum. J. Biol. Chem. 288, 16460–16475. doi: 10.1074/jbc.M113.450239
Pedersen, C. T., Loke, I., Lorentzen, A., Wolf, S., Kamble, M., Kristensen, S. K., et al. (2017). N-glycan maturation mutants in Lotus japonicus for basic and applied glycoprotein research. Plant J. 91, 394–407. doi: 10.1111/tpj.13570
Renna, L., Hanton, S., Stefano, G., Bortolotti, L., Misra, V., and Brandizzi, F. (2005). Identification and characterization of AtCASP, a plant transmembrane Golgi matrix protein. Plant Mol. Biol. 58, 109–122. doi: 10.1007/s11103-005-4618-4
Rips, S., Bentley, N., Jeong, I. S., Welch, J. L., von Schaewen, A., and Koiwa, H. (2014). Multiple N-glycans cooperate in the subcellular targeting and functioning of Arabidopsis KORRIGAN1. Plant Cell 26, 3792–3808. doi: 10.1105/tpc.114.129718
Runions, J., Brach, T., Kühner, S., and Hawes, C. (2006). Photoactivation of GFP reveals protein dynamics within the endoplasmic reticulum membrane. J. Exp. Bot. 57, 43–50. doi: 10.1093/jxb/eri289
Saint-Jore-Dupas, C., Nebenführ, A., Boulaflous, A., Follet-Gueye, M., Plasson, C., Hawes, C., et al. (2006). Plant N-glycan processing enzymes employ different targeting mechanisms for their spatial arrangement along the secretory pathway. Plant Cell 18, 3182–3200. doi: 10.1105/tpc.105.036400
Schneider, C. A., Rasband, W. S., and Eliceiri, K. W. (2012). NIH Image to ImageJ: 25 years of image analysis. Nat. Methods 9, 671–675. doi: 10.1038/nmeth.2089
Schoberer, J., Liebminger, E., Botchway, S. W., Strasser, R., and Hawes, C. (2013). Time-resolved fluorescence imaging reveals differential interactions of N-glycan processing enzymes across the Golgi stack in planta. Plant Physiol. 161, 1737–1754. doi: 10.1104/pp.112.210757
Schoberer, J., Liebminger, E., Vavra, U., Veit, C., Castilho, A., Dicker, M., et al. (2014). The transmembrane domain of N -acetylglucosaminyltransferase I is the key determinant for its Golgi subcompartmentation. Plant J. 80, 809–822. doi: 10.1111/tpj.12671
Schoberer, J., Vavra, U., Stadlmann, J., Hawes, C., Mach, L., Steinkellner, H., et al. (2009). Arginine/lysine residues in the cytoplasmic tail promote ER export of plant glycosylation enzymes. Traffic 10, 101–115. doi: 10.1111/j.1600-0854.2008.00841.x
Shen, J., Ding, Y., Gao, C., Rojo, E., and Jiang, L. (2014). N-linked glycosylation of AtVSR1 is important for vacuolar protein sorting in Arabidopsis. Plant J. 80, 977–992. doi: 10.1111/tpj.12696
Shibuya, N., Goldstein, I. J., Van Damme, E. J., and Peumans, W. J. (1988). Binding properties of a mannose-specific lectin from the snowdrop (Galanthus nivalis) bulb. J. Biol. Chem. 263, 728–734.
Soussillane, P., D’Alessio, C., Paccalet, T., Fitchette, A., Parodi, A., Williamson, R., et al. (2009). N-glycan trimming by glucosidase II is essential for Arabidopsis development. Glycoconj. J. 26, 597–607. doi: 10.1007/s10719-008-9201-1
Strasser, R. (2014). Biological significance of complex N-glycans in plants and their impact on plant physiology. Front. Plant Sci. 5:363. doi: 10.3389/fpls.2014.00363
Strasser, R. (2016). Plant protein glycosylation. Glycobiology 26, 926–939. doi: 10.1093/glycob/cww023
Strasser, R., Mucha, J., Schwihla, H., Altmann, F., Glössl, J., and Steinkellner, H. (1999). Molecular cloning and characterization of cDNA coding for beta1,2N-acetylglucosaminyltransferase I (GlcNAc-TI) from Nicotiana tabacum. Glycobiology 9, 779–785. doi: 10.1093/glycob/9.8.779
Su, W., Liu, Y., Xia, Y., Hong, Z., and Li, J. (2011). Conserved endoplasmic reticulum-associated degradation system to eliminate mutated receptor-like kinases in Arabidopsis. Proc. Natl. Acad. Sci. U.S.A. 108, 870–875. doi: 10.1073/pnas.1013251108
Teixeira, M. A., Rajewski, A., He, J., Castaneda, O. G., Litt, A., and Kaloshian, I. (2018). Classification and phylogenetic analyses of the Arabidopsis and tomato G-type lectin receptor kinases. BMC Genomics 19:239. doi: 10.1186/s12864-018-4606-0
Veit, C., Vavra, U., and Strasser, R. (2015). N-glycosylation and plant cell growth. Methods Mol. Biol. 1242, 183–194. doi: 10.1007/978-1-4939-1902-4_16
von Schaewen, A., Frank, J., and Koiwa, H. (2008). Role of complex N-glycans in plant stress tolerance. Plant Signal. Behav. 3, 871–873. doi: 10.4161/psb.3.10.6227
von Schaewen, A., Sturm, A., O’Neill, J., and Chrispeels, M. (1993). Isolation of a mutant Arabidopsis plant that lacks N-acetyl glucosaminyl transferase I and is unable to synthesize Golgi-modified complex N-linked glycans. Plant Physiol. 102, 1109–1118. doi: 10.1104/pp.102.4.1109
Wee, E., Sherrier, D., Prime, T., and Dupree, P. (1998). Targeting of active sialyltransferase to the plant Golgi apparatus. Plant Cell 10, 1759–1768. doi: 10.1105/tpc.10.10.1759
Zhang, M., Henquet, M., Chen, Z., Zhang, H., Zhang, Y., Ren, X., et al. (2009). LEW3, encoding a putative alpha-1,2-mannosyltransferase (ALG11) in N-linked glycoprotein, plays vital roles in cell-wall biosynthesis and the abiotic stress response in Arabidopsis thaliana. Plant J. 60, 983–999. doi: 10.1111/j.1365-313X.2009.04013.x
Keywords: endoplasmic reticulum, Golgi apparatus, protein glycosylation, N-glycosylation, glycoprotein, mannosidase
Citation: Veit C, König J, Altmann F and Strasser R (2018) Processing of the Terminal Alpha-1,2-Linked Mannose Residues From Oligomannosidic N-Glycans Is Critical for Proper Root Growth. Front. Plant Sci. 9:1807. doi: 10.3389/fpls.2018.01807
Received: 11 April 2018; Accepted: 20 November 2018;
Published: 06 December 2018.
Edited by:
Elisabeth Jamet, Université Toulouse III Paul Sabatier, FranceReviewed by:
Tomomichi Fujita, Hokkaido University, JapanBerit Ebert, The University of Melbourne, Australia
Copyright © 2018 Veit, König, Altmann and Strasser. This is an open-access article distributed under the terms of the Creative Commons Attribution License (CC BY). The use, distribution or reproduction in other forums is permitted, provided the original author(s) and the copyright owner(s) are credited and that the original publication in this journal is cited, in accordance with accepted academic practice. No use, distribution or reproduction is permitted which does not comply with these terms.
*Correspondence: Richard Strasser, richard.strasser@boku.ac.at