Characterization Techniques to Assess Recyclability in Dynamic Polymer Networks
- 1Department of Chemistry and Biochemistry, California Polytechnic State University, San Luis Obispo, CA, United States
- 2Department of Chemistry, Davidson College, Davidson, NC, United States
The inclusion of dynamic covalent bonds in cross-linked polymer networks enables material reprocessing towards a circular economy. Bond dynamicity in this class of materials, called covalent adaptable networks (CANs), is characterized by a mixture of spectroscopic chemical and bulk materials techniques. These characterization methods illustrate the interdisciplinary nature of the field, bridging fundamental chemical insights with engineering-driven applications. Here, we seek to describe and highlight the complementary nature and nuances of these methods with an emphasis on practical applications for newcomers to the field.
1 Introduction
In recent years, researchers have introduced dynamic covalent bonds into cross-linked polymer materials, enabling their reprocessing. These covalent cross-links become dynamic in response to stimuli including temperature, light, or mechanical activation that facilitate bond exchange. This results in a change to the network topology and the arrangement of crosslinks present in the material (Maeda et al., 2009; Wojtecki et al., 2010; Victor et al., 2018; Jiang et al., 2019; Huang et al., 2020; Samanta et al., 2021). These materials, also called covalent adaptable networks (CANs) aim to combine the mechanical robust-ness of cross-linked materials at their service temperatures with reprocessability previously only attributed to thermoplastics (Kloxin et al., 2010; Bowman and Kloxin, 2012; Kloxin and Bowman, 2013; Denissen et al., 2015b; Jin et al., 2019; Podgórski et al., 2020a; Liu et al., 2020; van Zee and Nicolaÿ, 2020; Zhang et al., 2021). Considerable academic interest has materialized in this area, resulting in numerous comprehensive reviews focused on dynamic bond chemistries in CANs and their mechanical properties. Additionally, undergraduate student populations are interested in sustainability and discussion of CANs is a timely addition to the undergraduate curriculum (Schneiderman et al., 2014; Chen et al., 2021). This mini-review aims to serve as an overview of CAN characterization techniques and emphasize how complementary chemical, thermal, and mechanical characterization techniques provide new insights.
Mechanistically, CANs are categorized by their exchange mechanism: dissociative or associative as shown in Figure 1. In a dissociative mechanism, a bond or cross-link needs to break before another one can be formed, causing an inherent decrease in cross-link density during bond exchange. Some examples of dissociative exchange reactions include: Diels-Alder additions (Chen et al., 2002; Peterson et al., 2010; Amato et al., 2013), boronate ester (Cromwell et al., 2015), reversible alkoxyamines (Otsuka, 2013; Sato et al., 2014), and reversible thiol-yne reactions (van Herck et al., 2020). Polyhydroxyurethane (Fortman et al., 2015), polyimine (Taynton et al., 2014), and polyimide exchanges (van Lijsebetten et al., 2021) have also been reported. In an associative mechanism, bonds are formed before a bond is broken, typically via the formation of an intermediate. These networks, also known as vitrimers (Montarnal et al., 2011), maintain cross-linking density throughout the exchange process. Their mechanical properties are theoretically preserved throughout bond rearrangement processes. Some chemistries that exhibit associative exchange mechanisms are transesterification reactions (Montarnal et al., 2011; Capelot et al., 2012), olefin metathesis (Lu and Guan, 2012), dioxaborolane exchange, (Röttger et al., 2017; Breuillac et al., 2019; Caffy and Nicolaÿ, 2019), silyl ether exchange (Nishimura et al., 2017; Tretbar et al., 2019), vinylogous urethane exchange (Denissen et al., 2015a; Denissen et al., 2017; Tellers et al., 2019), and thiol addition-elimination exchange (Ishibashi and Kalow, 2018; El-Zaatari et al., 2020).
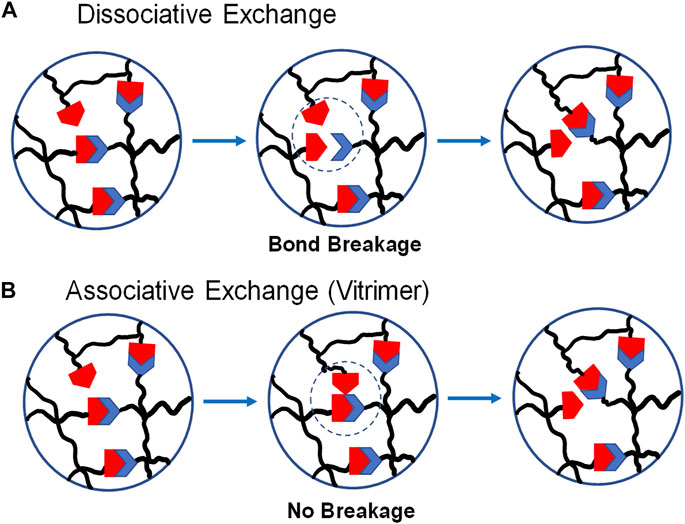
FIGURE 1. Covalent adaptable networks undergoing dynamic bond exchanges with (A) dissociative and (B) associative mechanisms.
2 Chemical Characterizations
To probe the nature of chemical bonding within CANs (e.g., bond dynamicity, decomposition) a range of techniques have been employed (Figure 2). These range from small molecule model “proof of concept” demonstrations of molecular exchange processes to bulk polymer characterization techniques.
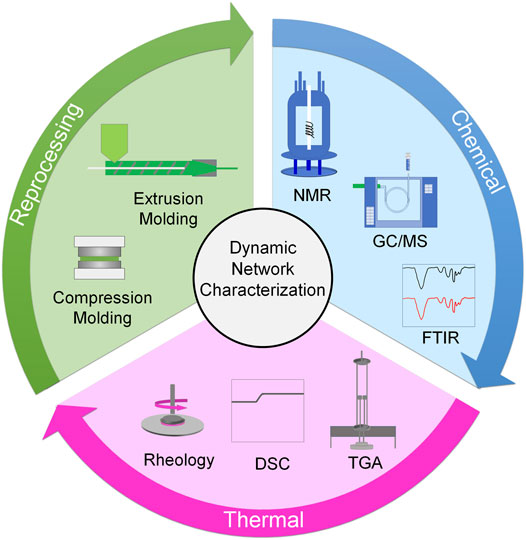
FIGURE 2. Characterization methods for covalent adaptable networks including chemical, thermal, and reprocessing techniques.
2.1 GC-MS
Gas Chromatography-Mass Spectrometry (GC-MS) is a versatile tool for characterizing samples containing relatively small molecules. In GC-MS, a heated column separates compounds present in a sample based on differences in boiling point and subsequently identifies them via a mass spectrometer. The GC, which can be coupled to a variety of detectors including MS and FID, also serves as a useful quantitation tool and can identify the concentration of components in a sample, with the aid of a calibration curve.
In CAN research, GC-MS has been used to monitor exchange reactions in small-molecule model compounds linked with the same bonds used in CANs. By linking R-groups of different mass and/or functionality to the dynamic bond being investigated, it is possible to identify when bonds have successfully been made dynamic. New compounds formed as a result of exchange processes will possess different retention times observable via GC-MS (Kim et al., 2021; Li et al., 2021a; Li et al., 2021b; Hernández et al., 2022). By monitoring the concentrations of A-A, B-B and B-A in samples subjected to a variety of conditions, it is possible to investigate the dynamic bond exchange reaction in great detail (Snyder et al., 2018; Li et al., 2021b). Bañales et al. were able to use GC-MS in this way to demonstrate the presence of a dynamic equilibrium between guanidine groups and several small-molecule amine compounds (Melchor Bañales and Larsen, 2020). GC-MS can also be coupled to other instruments, to analyze in more detail the byproducts of CAN degradation processes. For example, Li et al. used a coupled TGA-GCMS to monitor the thermal degradation products of thiourethanes in real-time (Li et al., 2019). This allowed them to determine that the decomposition products were primarily composed of their feedstock compounds. GC-MS is an incredibly powerful tool for investigating small-molecule reactions, and thus has excellent utility in small-molecule model compound studies of CANs.
2.2 NMR
Complementary to the experiments described above, model compound exchange can also be characterized via nuclear magnetic resonance (NMR). While GC-MS is limited to compounds with boiling points low enough to volatilize (<300°C), solution NMR probes exchange in higher molecular weight model compounds, provided that the species of interest possess resolvable chemical shifts. In some instances, both GC-MS and NMR model compound studies are employed to provide evidence of bond exchange. Small molecule model studies employing NMR have been performed to characterize and optimize bond exchange conditions for a variety of cross-linked systems including polyesters, polyurethanes, aminals, and guanidines (Chakma et al., 2020; Melchor Bañales and Larsen, 2020; Schoustra et al., 2021).
Small molecule model studies employing 1H NMR involve truncated analogues of the functional groups used in CAN synthesis. These are used to optimize exchange conditions (e.g. catalyst loading, temperature, etc.) that must be further optimized in polymer films (Melchor Bañales and Larsen, 2020). Additionally, model compound studies can be used to elucidate the reaction mechanism. For example, Delahaye et al. monitor transesterification reaction kinetics via 1H NMR for phthalate monoester compounds (Delahaye et al., 2019). Their NMR studies enabled classification of the reaction mechanism as associative or dissociative. Additionally, Schoustra et al. compared the kinetics of imine exchange via transimination and imine metathesis mechanisms for hydrocarbon and ethylene oxide-based polymers (Schoustra et al., 2021). These mechanistic studies led to inclusion of ethylene oxide groups in their final dynamic polymeric system. Due to the prevalence of variable temperature (VT) NMR instruments, a mixture of in situ and ex situ NMR studies are possible. Exchange reactions can be monitored in NMR tube reactions, or observed via NMR characterization of reaction aliquots. For example, Chakma et al. probe anilinium crosslinker dissociation equilibria at various temperatures. Reaction aliquots were added to CDCl3 prior to characterization (Chakma et al., 2020).
Although most work on CANs employ thermally activated exchange processes, there is growing interest in studying photo-activated exchange processes via NMR. Photo-activated exchange affords superior spatial control using light as the stimulus. Along these lines, Barsoum et al. demonstrated photoswitchable internal catalysis controlling exchange kinetics between a boronic ester and free diol where 1H VT-NMR is used to probe exchange kinetics (Barsoum and Kalow, 2021). These types of studies will enable incorporation of new photo-activated dynamic chemistries in CANs. Additionally, as in situ LED-NMR techniques evolve (Skubi et al., 2020) we suspect that in situ photoreactions monitoring bond exchange in model compounds will assist in the identification and development of new dynamic bonds that will be implemented in CANs.
Beyond model compound studies, NMR is used to characterize polymer products. Solution 1H NMR has been used to confirm successful polymerization of CANs from monomers (Huang et al., 2021). Other authors perform solid state NMR (ssNMR) experiments to confirm the successful formation and lack of undesirable degradation of their polymer material (Markwart et al., 2020). Additionally, ssNMR can be used to probe exchange processes in actual films. For example, Majumdar et al. used 31P ssNMR to probe β-hydroxyl-mediated transesterifications of hydroxyethyl phosphate triesters (Majumdar et al., 2021). Because bond exchange processes in model compound systems may differ from actual films, they probed bond exchange within actual polymer films.
2.3 FTIR
Fourier-Transform Infrared Spectroscopy (FTIR) has limited use in characterizing the evolution of bonds in covalent adaptable networks, but is primarily used to monitor polymer degradation. Gamardella et al. used FTIR to identify degradation reactions that occurred in their thiourethane CAN (Gamardella et al., 2020). Other groups, including Taplan et al., used ATR-FTIR to affirm that the chemical composition of their CAN did not change when recycled (He et al., 2021; Taplan et al., 2021).
Variable Temperature FTIR (VT-FTIR) probes how bonds present in a CAN change with temperature (Scheutz et al., 2019; Shi et al., 2020). Bongiardina et al. utilized VT-FTIR to examine the kinetics of bond formation in thiol-thioester reactions, by measuring the time required for the FTIR to show bond equilibration at a given temperature (Bongiardina et al., 2021). Additionally, Zhang et al. used VT ATR-FTIR to quantitate the Keq of several sterically distinct polyureas, using the Beer-Lambert law and an inert reference peak as an internal standard (Zhang and Rowan, 2017).
However, FTIR is only useful as a characterization technique for CANs when the change in functional groups is strongly detectable. This requires that the functional group change during the exchange process, and at a high enough concentration to give good resolution. For associative exchange mechanisms and some dissociative exchange mechanisms, the functional group does not significantly change during the exchange process. Thus, bond exchange cannot often be characterized via FTIR. Furthermore, the number of bonds engaged in dissociative exchange is often too low to quantitate reliably using VT-FTIR. In addition, FTIR characterization is not well-suited to solvent-borne exchange reactions, as the signal from exchange compounds is often drowned out by the solvent signal.
3 Bulk Mechanical and Thermal Characterizations
Many thermal and mechanical characterization techniques are applicable to CANs at temperatures below bond exchange activation (Figure 2). Most of these techniques are highlighted by Danielsen et al. (Danielsen et al., 2021). Of noted importance to CANs, is the ability to measure the glass transition temperature (Tg) and degradation temperature (Td) of the bulk polymer.
3.1 Tg Measurements
For conventionally cross-linked polymer networks, Tg represents a temperature below which the macromolecular structure of the polymer is “locked” and exhibits glass-like behavior. Above Tg, the polymer exhibits some mobility and is rubber-like.
Differential Scanning Calorimetry (DSC) is a common technique used to measure Tg’s of cross-linked polymer networks. Using a reference and the polymer sample, DSC monitors the amount of heat given to these two samples to find transition points. Tg is determined via a half-height or midline technique in the transition region. Multiple papers reporting Tg’s of their covalent adaptable network use DSC (Luo et al., 2018; Hao et al., 2020; Alraddadi et al., 2021; bin Rusayyis and Torkelson, 2021; Li et al., 2021b; Pronoitis et al., 2021; Taplan et al., 2021; Liguori and Hakkarainen, 2022). DSC has also been utilized to calculate degree of crystallization and melting temperature for crystalline CANs (Miao et al., 2020; Pronoitis et al., 2021).
Dynamic mechanical analysis (DMA) can also be used to calculate Tg in CANs (Giebler et al., 2020; Podgórski et al., 2020b; Ishibashi et al., 2021). This method quantifies how a material responds to an applied oscillatory force. During a temperature ramp, DMA measures elastic (storage modulus; E′) and viscous (loss modulus; E″) components of the polymer networks. The Tg of the material can be calculated by the inflection point of the storage modulus after it decreases during the temperature ramp, or by looking at the temperature at the peak of the Tan Delta curve. The Tan Delta represents the ratio of loss to storage modulus.
Slight differences in obtained Tg values between DSC and DMA have commonly been attributed to the difference in what each technique measures. While the DMA measurement pertains to changes in mechanical relaxation, DSC is sensitive to the material’s heat capacity changes. Thus, it is recommended that Tg values from both techniques be obtained if possible.
3.2 Td Measurements
Since many dynamic bonds in CANs are thermally activated, it is important to measure their degradation temperatures (Td) (Taplan et al., 2021; van Lijsebetten et al., 2021). Td is the temperature above which an irreversible change to the macromolecular structure of the polymer occurs (Danielsen et al., 2021). It is important that the operating temperature for CANs remains below Td to prevent chemical degradation of the material. Thermogravimetric Analysis (TGA) is used to determine Td by measuring change in mass as a function of increasing temperature under a controlled atmosphere. For neat (solvent-free) polymer networks, the temperature at which the network loses 5% mass is considered its thermal degradation temperature.
3.3 Assessing Recyclability
3.3.1 DMA vs. Rheology
Both DMA and rheology measure viscoelastic properties in polymer networks. The main difference between both instruments is that DMA utilizes compressive forces while rheology uses shear forces during testing (Meyvis et al., 2002). If the sample can be easily sheared (i.e., a material that has prominent viscous behavior to it), oscillatory shear rheology is a suitable technique since the sample can be sheared in between parallel plates without slippage. In cases where the sample has a more elastic behavior to it, where the sample cannot be easily sheared without fear of slippage or deformation, DMA is recommended.
3.3.2 Stress Relaxation
Stress relaxation experiments are rheological characterization experiments in which a polymer sample is subject to constant strain. The resulting stress is monitored over time via observation of the relaxation modulus (G(t)). During a stress relaxation event, G(t) decreases from an initial value, G0, to a lower plateau value, G∞. In many cases, stress relaxation is directly related to energy dissipation within a polymer. An observed decrease in stress often correlates with a topological reorganization of the cross-linked polymer and is an effective measure of bond dynamicity. In CANs, the relaxation behavior of the polymer can thus quantify both reprocessing ability and bulk exchange kinetics. In other words, the faster a polymer is able to dissipate energy when a constant strain is applied, the faster its theoretical reprocessing speed. The speed of the relaxation process can be defined in terms of a critical time constant known as the relaxation time, τ. In its simplest definition, τ is the time needed for the relaxation modulus (G(t)) to decrease to 1/e of the initial value (G0) (Chakma et al., 2020). This assumption is only valid if a material is considered Maxwellian, meaning it has a single relaxation process, which most CANs have been assumed to follow (Parada and Zhao, 2018). This assumption follows that the energy dissipation of the material can be traced back to a single relaxation event through activated bond exchange as the sole cause for network rearrangement. In such materials, τ can furthermore be related to the flow activation energy (Ea) of the polymer through an Arrhenius-type relationship (Brutman et al., 2014):
where R is the ideal gas constant, T is the temperature (in Kelvin), and A is a pre-exponential factor. The flow activation energy represents the energy required for polymer chain segment mobility and is used to assess the degree of difficulty with respect to material flow and informs on feasibility of reprocessability (Lou et al., 2019). Calculating both τ and Ea through stress relaxation experiments are critical to understanding flow behavior of CANs. Ea can be obtained by performing a linear regression to the plot of ln(τ) as a function of inverse temperature. The slope of this line equals Ea/R. It should be noted however, that if the CAN cannot be explained by a single Maxwell element, stress relaxation of these materials can be fitted to a Kohlrausch− Williams−Watts (KWW) which is a stretched exponential decay (Li et al., 2018).
Finally, creep measurements can be performed on CAN samples in order to assess their ability to permanently deform under applied mechanical stress These measurements typically take place through tension-film geometries and are loaded with constant stress, followed by sample length measurements after a certain amount of time (Li et al., 2018; bin Rusayyis and Torkelson, 2021).
3.3.3 Frequency Sweeps
Under small amplitude oscillatory shear, the storage (G′) and loss (G″) moduli are frequency dependent where they characterize the solid-like elastic and liquid-like viscous material responses respectively. At higher frequencies, the storage modulus dominates where G′ is greater than G″. This observation implies that the deformation energies are stored elastically over short time scales. As the frequency decreases, G″ approaches G′ until it reaches a crossover frequency, ωc, where G′(ωc) = G″(ωc). Beyond this crossover frequency, the loss modulus dominates where G″ is greater than G′ suggesting viscous storage of deformation energy. In an ideal Maxwell material, the relaxation time, τ, is inversely proportional to ωc
It is recommended that τ be calculated using both stress relaxation and frequency sweep measurements to ensure accuracy in quantifying flow behavior and recycling ability of CANs.
3.3.4 Dilatometry
Dilatometry takes advantage of the differences in volume occupied by a cross-linked thermoset material and a CAN with thermally activated dynamic bonds. Since permanently cross-linked networks occupy lower volumes than CANs, their expansion coefficient should be lower. Thus, changes in the expansion coefficient in CANs over a range of temperatures can indicate the temperature at which bond rearrangement is taking place. For CANs that undergo associative bond exchanges (i.e. vitrimers), a second temperature can be seen via dilatometry as the topology freezing transition temperature (Tv) (Yang et al., 2016; Liu et al., 2018). Below Tv, a polymer is mostly unaffected by the exchange reaction. Above Tv, the exchange reaction is favored and the polymer is able to be reprocessed and recycled. Tv can hence be considered as the minimum temperature required for reprocessing vitrimers. Methods for accurately measuring Tv are in the process of being determined within the field (Yang et al., 2019; Kaiser et al., 2020; Hubbard et al., 2021).
3.4 Reprocessing Techniques and Characterization
The ability to reprocess CANs while maintaining key physical properties of the virgin polymer sample is a defining feature of these materials (Figure 2). Typically, reprocessing is examined through compression molding, welding, and/or extrusion. After reprocessing is complete, the mechanical properties of the CAN before and after reprocessing are assessed via FTIR or Raman spectroscopy (van Herck et al., 2020) and/or DMA/rheology (Ishibashi and Kalow, 2018). Comparing stress-strain plots before and after multi-cycle reprocessing is furthermore recommended (Zheng et al., 2018; Song et al., 2021; Zeng et al., 2021).
3.4.1 Compression Molding
During compression molding (Snyder et al., 2018), CANs are cut into small pieces and placed in a mold. Next, the samples are placed between two plates in a hot press at controlled temperature and pressure. The chosen temperature for reprocessing has to be high enough to activate dynamic bond chemistry, but low enough to avoid degradation temperatures (Td). Once the sample has reached the desired temperature, it is compressed between the plates at high pressures. After a certain amount of time, which is usually correlated with the relaxation time, τ, the sample is released, cooled down, and removed at room temperature.
3.4.2 Welding
During welding (Yu et al., 2016; He et al., 2018), two independent CAN films are typically overlapped and gently pressed together. The samples are then heated at a temperature below the degradation temperature. The bond exchange reaction occurs at the interface through chain crossing between the two films, causing the welding of the two films into one.
3.4.3 Extrusion
Extrusion of polymers is a highly utilized manufacturing process to shape plastics for their desired end application while simultaneously enriching them with additives if required. In this process, the material is melted and formed continuously. Most examples of extrusion molding of CANs have involved small scale molding through a mini extruder or a twin-screw extruder at high temperatures (Yue et al., 2019; Sheppard et al., 2020; Swartz et al., 2021).
4 Conclusion and Outlook
Cross-linked polymer networks are ubiquitous to daily life but are impeded by our inability to repair or recycle these materials. This has contributed to a global environmental crisis. The utility of dynamic bonds in polymer networks is emerging as an essential combatant to plastic waste, revolutionizing the way traditional polymers are viewed. This mini-review focuses on techniques used to characterize properties of covalent adaptable networks which contain dynamic bonds on the chemical, thermal, mechanical, and reprocessing levels. While all characterization techniques could not be covered, we hope that this review will serve as a starting point for students, academics, and scientists eager to develop their understanding of these unique class of materials.
Author Contributions
AL, BE-Z, and LH wrote, edited, and approved of the final manuscript. AL and LH worked on the chemical characterizations section. BE-Z drafted figures and worked on the thermal and mechanical properties section.
Funding
AL was funded by the Bill and Linda Frost Summer Undergraduate Research Program and the Frost Academic Year Undergraduate Research Program.
Conflict of Interest
The authors declare that the research was conducted in the absence of any commercial or financial relationships that could be construed as a potential conflict of interest.
Publisher’s Note
All claims expressed in this article are solely those of the authors and do not necessarily represent those of their affiliated organizations, or those of the publisher, the editors and the reviewers. Any product that may be evaluated in this article, or claim that may be made by its manufacturer, is not guaranteed or endorsed by the publisher.
Acknowledgments
AL and LH acknowledge the Kenneth N. Edwards Western Coatings Technology Center.
References
Alraddadi, M. A., Chiaradia, V., Stubbs, C. J., Worch, J. C., and Dove, A. P. (2021). Renewable and Recyclable Covalent Adaptable Networks Based on Bio-Derived Lipoic Acid. Polym. Chem. 12, 5796–5802. doi:10.1039/D1PY00754H
Amato, D. N., Strange, G. A., Swanson, J. P., Chavez, A. D., Roy, S. E., Varney, K. L., et al. (2013). Synthesis and Evaluation of Thermally-Responsive Coatings Based upon Diels-Alder Chemistry and Renewable Materials. Polym. Chem. 5, 69–76. doi:10.1039/C3PY01024D
Barsoum, D., and Kalow, J. (2021). Remote-Controlled Exchange Rates by Photoswitchable Internal Catalysis of Dynamic Covalent Bonds. ChemRxiv. doi:10.26434/CHEMRXIV-2021-LWK72
bin Rusayyis, M. A., and Torkelson, J. M. (2021). Reprocessable Covalent Adaptable Networks with Excellent Elevated-Temperature Creep Resistance: Facilitation by Dynamic, Dissociative Bis(hindered Amino) Disulfide Bonds. Polym. Chem. 12, 2760–2771. doi:10.1039/D1PY00187F
Bongiardina, N. J., Long, K. F., Podgórski, M., and Bowman, C. N. (2021). Substituted Thiols in Dynamic Thiol-Thioester Reactions. Macromolecules 54, 8341–8351. doi:10.1021/ACS.MACROMOL.1C00649
Bowman, C. N., and Kloxin, C. J. (2012). Covalent Adaptable Networks: Reversible Bond Structures Incorporated in Polymer Networks. Angew. Chem. Int. Ed. 51, 4272–4274. doi:10.1002/anie.201200708
Breuillac, A., Kassalias, A., and Nicolaÿ, R. (2019). Polybutadiene Vitrimers Based on Dioxaborolane Chemistry and Dual Networks with Static and Dynamic Cross-Links. Macromolecules 52, 7102–7113. doi:10.1021/ACS.MACROMOL.9B01288
Brutman, J. P., Delgado, P. A., and Hillmyer, M. A. (2014). Polylactide Vitrimers. ACS Macro Lett. 3, 607–610. doi:10.1021/MZ500269W
Caffy, F., and Nicolaÿ, R. (2019). Transformation of Polyethylene into a Vitrimer by Nitroxide Radical Coupling of a Bis-Dioxaborolane. Polym. Chem. 10, 3107–3115. doi:10.1039/C9PY00253G
Capelot, M., Unterlass, M. M., Tournilhac, F., and Leibler, L. (2012). Catalytic Control of the Vitrimer Glass Transition. ACS Macro Lett. 1, 789–792. doi:10.1021/mz300239f
Chakma, P., Morley, C. N., Sparks, J. L., and Konkolewicz, D. (2020). Exploring How Vitrimer-Like Properties Can Be Achieved from Dissociative Exchange in Anilinium Salts. Macromolecules 53, 1233–1244. doi:10.1021/ACS.MACROMOL.0C00120
Chen, Q., Yang, Y., Yu, Y., and Xu, H. (2021). "Reprocessable Thermosets": Synthesis and Characterization of Vitrimer in the Undergraduate Lab Course. J. Chem. Educ. 98, 1429–1435. doi:10.1021/ACS.JCHEMED.0C01289
Chen, X., Dam, M. A., Ono, K., Mal, A., Shen, H., Nutt, S. R., et al. (2002). A Thermally Re-Mendable Cross-Linked Polymeric Material. Science (1979) 295, 1698–1702. doi:10.1126/SCIENCE.1065879
Cromwell, O. R., Chung, J., and Guan, Z. (2015). Malleable and Self-Healing Covalent Polymer Networks through Tunable Dynamic Boronic Ester Bonds. J. Am. Chem. Soc. 137, 6492–6495. doi:10.1021/JACS.5B03551
Danielsen, S. P. O., Beech, H. K., Wang, S., El-Zaatari, B. M., Wang, X., Sapir, L., et al. (2021). Molecular Characterization of Polymer Networks. Chem. Rev. 121, 5042–5092. doi:10.1021/ACS.CHEMREV.0C01304
Delahaye, M., Winne, J. M., and du Prez, F. E. (2019). Internal Catalysis in Covalent Adaptable Networks: Phthalate Monoester Transesterification as a Versatile Dynamic Cross-Linking Chemistry. J. Am. Chem. Soc. 141, 15277–15287. doi:10.1021/JACS.9B07269
Denissen, W., Droesbeke, M., Nicolaÿ, R., Leibler, L., Winne, J. M., and du Prez, F. E. (2017). Chemical Control of the Viscoelastic Properties of Vinylogous Urethane Vitrimers. Nat. Commun. 8 (1), 14857. doi:10.1038/ncomms14857
Denissen, W., Rivero, G., Nicolaÿ, R., Leibler, L., Winne, J. M., and du Prez, F. E. (2015a). Vinylogous Urethane Vitrimers. Adv. Funct. Mat. 25, 2451–2457. doi:10.1002/ADFM.201404553
Denissen, W., Winne, J. M., and du Prez, F. E. (2015b). Vitrimers: Permanent Organic Networks with Glass-Like Fluidity. Chem. Sci. 7, 30–38. doi:10.1039/C5SC02223A
El-Zaatari, B. M., Ishibashi, J. S. A., and Kalow, J. A. (2020). Cross-Linker Control of Vitrimer Flow. Polym. Chem. 11, 5339–5345. doi:10.1039/D0PY00233J
Fortman, D. J., Brutman, J. P., Cramer, C. J., Hillmyer, M. A., and Dichtel, W. R. (2015). Mechanically Activated, Catalyst-Free Polyhydroxyurethane Vitrimers. J. Am. Chem. Soc. 137, 14019–14022. doi:10.1021/JACS.5B08084
Gamardella, F., Muñoz, S., de la Flor, S., Ramis, X., and Serra, A. (2020). Recyclable Organocatalyzed Poly(Thiourethane) Covalent Adaptable Networks. Polymers 12 (12), 2913. doi:10.3390/POLYM12122913
Giebler, M., Sperling, C., Kaiser, S., Duretek, I., and Schlögl, S. (2020). Epoxy-Anhydride Vitrimers from Aminoglycidyl Resins with High Glass Transition Temperature and Efficient Stress Relaxation. Polymers (Basel) 12, 1148. doi:10.3390/POLYM12051148
Hao, C., Liu, T., Zhang, S., Liu, W., Shan, Y., and Zhang, J. (2020). Triethanolamine-Mediated Covalent Adaptable Epoxy Network: Excellent Mechanical Properties, Fast Repairing, and Easy Recycling. Macromolecules 53, 3110–3118. doi:10.1021/ACS.MACROMOL.9B02243
He, X., Hanzon, D. W., and Yu, K. (2018). Cyclic Welding Behavior of Covalent Adaptable Network Polymers. J. Polym. Sci. Part B Polym. Phys. 56, 402–413. doi:10.1002/POLB.24553
He, X., Shi, X., Chung, C., Lei, Z., Zhang, W., and Yu, K. (2021). A Sustainable Manufacturing Method of Thermoset Composites Based on Covalent Adaptable Network Polymers. Compos. Part B Eng. 221, 109004. doi:10.1016/J.COMPOSITESB.2021.109004
Hernández, A., Houck, H. A., Elizalde, F., Guerre, M., Sardon, H., and du Prez, F. E. (2022). Internal Catalysis on the Opposite Side of the Fence in Non-Isocyanate Polyurethane Covalent Adaptable Networks. Eur. Polym. J. 168, 111100. doi:10.1016/J.EURPOLYMJ.2022.111100
Huang, S., Kong, X., Xiong, Y., Zhang, X., Chen, H., Jiang, W., et al. (2020). An Overview of Dynamic Covalent Bonds in Polymer Material and Their Applications. Eur. Polym. J. 141, 110094. doi:10.1016/J.EURPOLYMJ.2020.110094
Huang, S., Shen, Y., Bisoyi, H. K., Tao, Y., Liu, Z., Wang, M., et al. (2021). Covalent Adaptable Liquid Crystal Networks Enabled by Reversible Ring-Opening Cascades of Cyclic Disulfides. J. Am. Chem. Soc. 143, 12543–12551. doi:10.1021/JACS.1C03661
Hubbard, A. M., Ren, Y., Konkolewicz, D., Sarvestani, A., Picu, C. R., Kedziora, G. S., et al. (2021). Vitrimer Transition Temperature Identification: Coupling Various Thermomechanical Methodologies. ACS Appl. Polym. Mat. 3, 1756–1766. doi:10.1021/ACSAPM.0C01290
Ishibashi, J. S. A., and Kalow, J. A. (2018). Vitrimeric Silicone Elastomers Enabled by Dynamic Meldrum's Acid-Derived Cross-Links. ACS Macro Lett. 7, 482–486. doi:10.1021/ACSMACROLETT.8B00166
Ishibashi, J. S. A., Pierce, I. C., Chang, A. B., Zografos, A., El-Zaatari, B. M., Fang, Y., et al. (2021). Mechanical and Structural Consequences of Associative Dynamic Cross-Linking in Acrylic Diblock Copolymers. Macromolecules 54, 3972–3986. doi:10.1021/acs.macromol.0c02744
Jiang, Z., Bhaskaran, A., Aitken, H. M., Shackleford, I. C. G., Connal, L. A., Jiang, Z., et al. (2019). Using Synergistic Multiple Dynamic Bonds to Construct Polymers with Engineered Properties. Macromol. Rapid Commun. 40, 1900038. doi:10.1002/MARC.201900038
Jin, Y., Lei, Z., Taynton, P., Huang, S., and Zhang, W. (2019). Malleable and Recyclable Thermosets: The Next Generation of Plastics. Matter 1, 1456–1493. doi:10.1016/j.matt.2019.09.004
Kaiser, S., Novak, P., Giebler, M., Gschwandl, M., Novak, P., Pilz, G., et al. (2020). The Crucial Role of External Force in the Estimation of the Topology Freezing Transition Temperature of Vitrimers by Elongational Creep Measurements. Polymer 204, 122804. doi:10.1016/J.POLYMER.2020.122804
Kim, H., Cha, I., Yoon, Y., Cha, B. J., Yang, J., Kim, Y. D., et al. (2021). Facile Mechanochemical Synthesis of Malleable Biomass-Derived Network Polyurethanes and Their Shape-Memory Applications. ACS Sustain. Chem. Eng. 9, 6952–6961. doi:10.1021/ACSSUSCHEMENG.1C00390
Kloxin, C. J., and Bowman, C. N. (2013). Covalent Adaptable Networks: Smart, Reconfigurable and Responsive Network Systems. Chem. Soc. Rev. 42, 7161–7173. doi:10.1039/c3cs60046g
Kloxin, C. J., Scott, T. F., Adzima, B. J., and Bowman, C. N. (2010). Covalent Adaptable Networks (CANs): A Unique Paradigm in Cross-Linked Polymers. Macromolecules 43, 2643–2653. doi:10.1021/ma902596s
Li, L., Chen, X., Jin, K., and Torkelson, J. M. (2018). Vitrimers Designed Both to Strongly Suppress Creep and to Recover Original Cross-Link Density after Reprocessing: Quantitative Theory and Experiments. Macromolecules 51, 5537–5546. doi:10.1021/ACS.MACROMOL.8B00922
Li, L., Chen, X., and Torkelson, J. M. (2019). Reprocessable Polymer Networks via Thiourethane Dynamic Chemistry: Recovery of Cross-Link Density after Recycling and Proof-of-Principle Solvolysis Leading to Monomer Recovery. Macromolecules 52, 8207–8216. doi:10.1021/ACS.MACROMOL.9B01359
Li, Q., Ma, S., Li, P., Wang, B., Feng, H., Lu, N., et al. (2021a). Biosourced Acetal and Diels-Alder Adduct Concurrent Polyurethane Covalent Adaptable Network. Macromolecules 54, 1742–1753. doi:10.1021/ACS.MACROMOL.0C02699
Li, Q., Ma, S., Li, P., Wang, B., Yu, Z., Feng, H., et al. (2021b). Fast Reprocessing of Acetal Covalent Adaptable Networks with High Performance Enabled by Neighboring Group Participation. Macromolecules 54, 8423–8434. doi:10.1021/ACS.MACROMOL.1C01046
Liguori, A., and Hakkarainen, M. (2022). Designed from Biobased Materials for Recycling: Imine‐Based Covalent Adaptable Networks. Macromol. Rapid Commun., 2100816. doi:10.1002/MARC.202100816
Liu, T., Zhao, B., and Zhang, J. (2020). Recent Development of Repairable, Malleable and Recyclable Thermosetting Polymers through Dynamic Transesterification. Polymer 194, 122392. doi:10.1016/J.POLYMER.2020.122392
Liu, Y., Tang, Z., Chen, Y., Zhang, C., and Guo, B. (2018). Engineering of β-Hydroxyl Esters into Elastomer-Nanoparticle Interface toward Malleable, Robust, and Reprocessable Vitrimer Composites. ACS Appl. Mat. Interfaces 10, 2992–3001. doi:10.1021/ACSAMI.7B17465
Lou, Y., Lei, Q., and Wu, G. (2019). Research on Polymer Viscous Flow Activation Energy and Non-newtonian Index Model Based on Feature Size. Adv. Polym. Technol. 2019, 1–11. doi:10.1155/2019/1070427
Lu, Y.-X., and Guan, Z. (2012). Olefin Metathesis for Effective Polymer Healing via Dynamic Exchange of Strong Carbon-Carbon Double Bonds. J. Am. Chem. Soc. 134, 14226–14231. doi:10.1021/JA306287S
Luo, C., Lei, Z., Mao, Y., Shi, X., Zhang, W., and Yu, K. (2018). Chemomechanics in the Moisture-Induced Malleability of Polyimine-Based Covalent Adaptable Networks. Macromolecules 51, 9825–9838. doi:10.1021/ACS.MACROMOL.8B02046
Maeda, T., Otsuka, H., and Takahara, A. (2009). Dynamic Covalent Polymers: Reorganizable Polymers with Dynamic Covalent Bonds. Prog. Polym. Sci. 34, 581–604. doi:10.1016/J.PROGPOLYMSCI.2009.03.001
Majumdar, S., Mezari, B., Zhang, H., van Aart, J., van Benthem, R. A. T. M., Heuts, J. P. A., et al. (2021). Efficient Exchange in a Bioinspired Dynamic Covalent Polymer Network via a Cyclic Phosphate Triester Intermediate. Macromolecules 54, 7955–7962. doi:10.1021/acs.macromol.1c01504
Markwart, J. C., Battig, A., Urbaniak, T., Haag, K., Koschek, K., Schartel, B., et al. (2020). Intrinsic Flame Retardant Phosphonate-Based Vitrimers as a Recyclable Alternative for Commodity Polymers in Composite Materials. Polym. Chem. 11, 4933–4941. doi:10.1039/D0PY00275E
Melchor Bañales, A. J., and Larsen, M. B. (2020). Thermal Guanidine Metathesis for Covalent Adaptable Networks. ACS Macro Lett. 9, 937–943. doi:10.1021/ACSMACROLETT.0C00352
Meyvis, T. K. L., Stubbe, B. G., van Steenbergen, M. J., Hennink, W. E., de Smedt, S. C., and Demeester, J. (2002). A Comparison between the Use of Dynamic Mechanical Analysis and Oscillatory Shear Rheometry for the Characterisation of Hydrogels. Int. J. Pharm. 244, 163–168. doi:10.1016/S0378-5173(02)00328-9
Miao, W., Zou, W., Jin, B., Ni, C., Zheng, N., Zhao, Q., et al. (2020). On Demand Shape Memory Polymer via Light Regulated Topological Defects in a Dynamic Covalent Network. Nat. Commun. 11 (1), 1–8. doi:10.1038/s41467-020-18116-1
Montarnal, D., Capelot, M., Tournilhac, F., and Leibler, L. (2011). Silica-Like Malleable Materials from Permanent Organic Networks. Science (1979) 334, 965–968. doi:10.1126/science.1212648
Nishimura, Y., Chung, J., Muradyan, H., and Guan, Z. (2017). Silyl Ether as a Robust and Thermally Stable Dynamic Covalent Motif for Malleable Polymer Design. J. Am. Chem. Soc. 139, 14881–14884. doi:10.1021/JACS.7B08826
Otsuka, H. (2013). Reorganization of Polymer Structures Based on Dynamic Covalent Chemistry: Polymer Reactions by Dynamic Covalent Exchanges of Alkoxyamine Units. Polym. J. 45 (9), 879–891. doi:10.1038/pj.2013.17
Parada, G. A., and Zhao, X. (2018). Ideal Reversible Polymer Networks. Soft Matter 14, 5186–5196. doi:10.1039/C8SM00646F
Peterson, A. M., Jensen, R. E., and Palmese, G. R. (2010). Room-Temperature Healing of a Thermosetting Polymer Using the Diels−Alder Reaction. ACS Appl. Mat. Interfaces 2, 1141–1149. doi:10.1021/AM9009378
Podgórski, M., Fairbanks, B. D., Kirkpatrick, B. E., McBride, M., Martinez, A., Dobson, A., et al. (2020a). Toward Stimuli‐Responsive Dynamic Thermosets through Continuous Development and Improvements in Covalent Adaptable Networks (CANs). Adv. Mat. 32, 1906876. doi:10.1002/ADMA.201906876
Podgórski, M., Spurgin, N., Mavila, S., and Bowman, C. N. (2020b). Mixed Mechanisms of Bond Exchange in Covalent Adaptable Networks: Monitoring the Contribution of Reversible Exchange and Reversible Addition in Thiol-Succinic Anhydride Dynamic Networks. Polym. Chem. 11, 5365–5376. doi:10.1039/D0PY00091D
Pronoitis, C., Hakkarainen, M., and Odelius, K. (2021). Long-Chain Polyamide Covalent Adaptable Networks Based on Renewable Ethylene Brassylate and Disulfide Exchange. Polym. Chem. 12, 5668–5678. doi:10.1039/D1PY00811K
Röttger, M., Domenech, T., van der Weegen, R., Breuillac, A., Nicolaÿ, R., and Leibler, L. (2017). High-Performance Vitrimers from Commodity Thermoplastics through Dioxaborolane Metathesis. Science (1979) 356, 62–65. doi:10.1126/SCIENCE.AAH5281
Samanta, S., Kim, S., Saito, T., and Sokolov, A. P. (2021). Polymers with Dynamic Bonds: Adaptive Functional Materials for a Sustainable Future. J. Phys. Chem. B 125, 9389–9401. doi:10.1021/ACS.JPCB.1C03511
Sato, T., Amamoto, Y., Ohishi, T., Higaki, Y., Takahara, A., and Otsuka, H. (2014). Radical Crossover Reactions of a Dynamic Covalent Polymer Brush for Reversible Hydrophilicity Control. Polymer 55, 4586–4592. doi:10.1016/J.POLYMER.2014.07.010
Scheutz, G. M., Lessard, J. J., Sims, M. B., and Sumerlin, B. S. (2019). Adaptable Crosslinks in Polymeric Materials: Resolving the Intersection of Thermoplastics and Thermosets. J. Am. Chem. Soc. 141, 16181–16196. doi:10.1021/JACS.9B07922
Schneiderman, D. K., Gilmer, C., Wentzel, M. T., Martello, M. T., Kubo, T., and Wissinger, J. E. (2014). Sustainable Polymers in the Organic Chemistry Laboratory: Synthesis and Characterization of a Renewable Polymer from δ-Decalactone and L-Lactide. J. Chem. Educ. 91, 131–135. doi:10.1021/ED400185U/
Schoustra, S. K., Groeneveld, T., and Smulders, M. M. J. (2021). The Effect of Polarity on the Molecular Exchange Dynamics in Imine-Based Covalent Adaptable Networks. Polym. Chem. 12, 1635–1642. doi:10.1039/D0PY01555E
Sheppard, D. T., Jin, K., Hamachi, L. S., Dean, W., Fortman, D. J., Ellison, C. J., et al. (2020). Reprocessing Postconsumer Polyurethane Foam Using Carbamate Exchange Catalysis and Twin-Screw Extrusion. ACS Cent. Sci. 6, 921–927. doi:10.1021/ACSCENTSCI.0C00083
Shi, X., Soule, D., Mao, Y., Yakacki, C., Lu, H., and Yu, K. (2020). A Multiscale Chemomechanics Theory for the Solvent - Assisted Recycling of Covalent Adaptable Network Polymers. J. Mech. Phys. Solids 138, 103918. doi:10.1016/J.JMPS.2020.103918
Skubi, K. L., Swords, W. B., Hofstetter, H., and Yoon, T. P. (2020). LED‐NMR Monitoring of an Enantioselective Catalytic [2+2] Photocycloaddition. ChemPhotoChem 4, 685–690. doi:10.1002/CPTC.202000094
Snyder, R. L., Fortman, D. J., de Hoe, G. X., Hillmyer, M. A., and Dichtel, W. R. (2018). Reprocessable Acid-Degradable Polycarbonate Vitrimers. Macromolecules 51, 389–397. doi:10.1021/ACS.MACROMOL.7B02299
Song, Z., Wang, Z., and Cai, S. (2021). Mechanics of Vitrimer with Hybrid Networks. Mech. Mater. 153, 103687. doi:10.1016/J.MECHMAT.2020.103687
Swartz, J. L., Sheppard, D. T., Haugstad, G., and Dichtel, W. R. (2021). Blending Polyurethane Thermosets Using Dynamic Urethane Exchange. Macromolecules 54, 11126–11133. doi:10.1021/ACS.MACROMOL.1C01910
Taplan, C., Guerre, M., and du Prez, F. E. (2021). Covalent Adaptable Networks Using β-Amino Esters as Thermally Reversible Building Blocks. J. Am. Chem. Soc. 143, 9140–9150. doi:10.1021/JACS.1C03316
Taynton, P., Yu, K., Shoemaker, R. K., Jin, Y., Qi, H. J., and Zhang, W. (2014). Heat- or Water-Driven Malleability in a Highly Recyclable Covalent Network Polymer. Adv. Mat. 26, 3938–3942. doi:10.1002/ADMA.201400317
Tellers, J., Pinalli, R., Soliman, M., Vachon, J., and Dalcanale, E. (2019). Reprocessable Vinylogous Urethane Cross-Linked Polyethylene via Reactive Extrusion. Polym. Chem. 10, 5534–5542. doi:10.1039/C9PY01194C
Tretbar, C. A., Neal, J. A., and Guan, Z. (2019). Direct Silyl Ether Metathesis for Vitrimers with Exceptional Thermal Stability. J. Am. Chem. Soc. 141, 16595–16599. doi:10.1021/JACS.9B08876
van Herck, N., Maes, D., Unal, K., Guerre, M., Winne, J. M., and du Prez, F. E. (2020). Covalent Adaptable Networks with Tunable Exchange Rates Based on Reversible Thiol-Yne Cross‐Linking. Angew. Chem. Int. Ed. 59, 3609–3617. doi:10.1002/ANIE.201912902
van Lijsebetten, F., Spiesschaert, Y., Winne, J. M., and du Prez, F. E. (2021). Reprocessing of Covalent Adaptable Polyamide Networks through Internal Catalysis and Ring-Size Effects. J. Am. Chem. Soc. 143, 15834–15844. doi:10.1021/JACS.1C07360
van Zee, N. J., and Nicolaÿ, R. (2020). Vitrimers: Permanently Crosslinked Polymers with Dynamic Network Topology. Prog. Polym. Sci. 104, 101233. doi:10.1016/J.PROGPOLYMSCI.2020.101233
Victor, K., Boris, L., Athina, G., Anthi, P., Marija, S., Marina, K., et al. (2018). Design, Synthesis and Antimicrobial Activity of Usnic Acid Derivatives. Med. Chem. Commun. 9, 870–882. doi:10.1039/C8MD00076J
Wojtecki, R. J., Meador, M. A., and Rowan, S. J. (2010). Using the Dynamic Bond to Access Macroscopically Responsive Structurally Dynamic Polymers. Nat. Mater 10 (1), 14–27. doi:10.1038/nmat2891
Yang, Y., Zhang, S., Zhang, X., Gao, L., Wei, Y., and Ji, Y. (2019). Detecting Topology Freezing Transition Temperature of Vitrimers by AIE Luminogens. Nat. Commun. 10 (1), 3165. doi:10.1038/s41467-019-11144-6
Yang, Z., Wang, Q., and Wang, T. (2016). Dual-Triggered and Thermally Reconfigurable Shape Memory Graphene-Vitrimer Composites. ACS Appl. Mat. Interfaces 8, 21691–21699. doi:10.1021/ACSAMI.6B07403/
Yu, K., Shi, Q., Li, H., Jabour, J., Yang, H., Dunn, M. L., et al. (2016). Interfacial Welding of Dynamic Covalent Network Polymers. J. Mech. Phys. Solids 94, 1–17. doi:10.1016/J.JMPS.2016.03.009
Yue, L., Bonab, V. S., Yuan, D., Patel, A., Karimkhani, V., Manas‐Zloczower, I., et al. (2019). Vitrimerization: A Novel Concept to Reprocess and Recycle Thermoset Waste via Dynamic Chemistry. Glob. Challenges 3, 1800076. doi:10.1002/GCH2.201800076
Zeng, Y., Yang, W., Liu, S., Shi, X., Xi, A., and Zhang, F. (2021). Dynamic Semi IPNs with Duple Dynamic Linkers: Self-Healing, Reprocessing, Welding, and Shape Memory Behaviors. Polymers 13 (11), 1679. doi:10.3390/POLYM13111679
Zhang, L., and Rowan, S. J. (2017). Effect of Sterics and Degree of Cross-Linking on the Mechanical Properties of Dynamic Poly(alkylurea-Urethane) Networks. Macromolecules 50, 5051–5060. doi:10.1021/ACS.MACROMOL.7B01016
Zhang, Y., Zhang, L., Yang, G., Yao, Y., Wei, X., Pan, T., et al. (2021). Recent Advances in Recyclable Thermosets and Thermoset Composites Based on Covalent Adaptable Networks. J. Mater. Sci. Technol. 92, 75–87. doi:10.1016/J.JMST.2021.03.043
Keywords: covalent adaptable network, dynamic covalent bond, characterization, recyclability, reprocessing, vitrimer, instrumentation
Citation: Lagron AB, El-Zaatari BM and Hamachi LS (2022) Characterization Techniques to Assess Recyclability in Dynamic Polymer Networks. Front. Mater. 9:915296. doi: 10.3389/fmats.2022.915296
Received: 07 April 2022; Accepted: 06 May 2022;
Published: 23 May 2022.
Edited by:
Andrea Dorigato, University of Trento, ItalyReviewed by:
Zepeng Lei, University of Colorado Boulder, United StatesMaciej Podgorski, Marie Curie-Sklodowska University, Poland
Copyright © 2022 Lagron, El-Zaatari and Hamachi. This is an open-access article distributed under the terms of the Creative Commons Attribution License (CC BY). The use, distribution or reproduction in other forums is permitted, provided the original author(s) and the copyright owner(s) are credited and that the original publication in this journal is cited, in accordance with accepted academic practice. No use, distribution or reproduction is permitted which does not comply with these terms.
*Correspondence: Bassil M. El-Zaatari, baelzaatari@davidson.edu; Leslie S. Hamachi, hamachi@calpoly.edu